Become a site member today and access 100's of fantastic biology resources, including interactive workbooks with detailed answers, quick revision sheets to reinforce key concepts and revision videos to help you learn key concepts quickly and effectively.
Diploid - What it means to be 2n
Nucleotides and Polynucleotides
DNA - The Structure and brief history of DNA
RNA - The Structure of RNA
mRNA and tRNA
Genes and non-coding DNA
Gene Mutations (substitutions and deletions).
Recombinant DNA (and the central dogma of biology)
Reverse Transcriptase
PCR - The Polymerase Chain Reaction
DNA profiling using VNTR's (aka genetic fingerprinting)
Population Genetics: The Hardy-Wienberg Equation
Genetics
Genetics is the study of heredity (the passing on of characteristics from one generation to the next). Genetics is concerned with a few key questions, but primarily the question
"Why do organisms look almost, but not exactly, like their parents?"
Genetics can be subdivided into 3 main areas: -
1. Mendelian Genetics (Classical Genetics). Mendelian Genetics (pioneered by Gregor Mendel: 1822-1884) is the study of heredity at the whole organism level by investigating how particular characteristics are inherited - it is in classical genetics where you learn all about Punnet Squares and investigating monohybrid and dihybrid inheritance. You can recap simple punnet squares and their application in population genetics in the Hardy-Weinberg lessons.
2. Molecular Genetics (Molecular Biology), is the study of heredity at the molecular level, thus, molecular genetics if primarily concerned with the structure, function and properties of DNA and nucleic acids. Molecular genetics also includes genetic engineering, molecular cloning, biotechnology and a whole host of cool cell biology stuff - like PCR and Genetic Fingerprinting.
3. Population Genetics, is the study of genetic differences within species and between species, including how species evolve by natural selection. Population genetics utilises statistical models (like the Hardy-Weinberg equilibrium) to investigate how characteristics evolve through populations.
In a previous section we learned all about nucleic acids: - DNA, RNA, mRNA, tRNA and also learned a bit about they key players involved in the discovery of DNA, important figures such as Crick, Watson, Franklin, Wilkins, and Chargaff…
in this section we move on from the structure of nucleic acids and take a look at how DNA forms chromosomes, the eukaryotic cell cycle, Mitosis, the meaning of diploid, meiosis, and gene mutations.
DNA molecules are pretty big polynucleotides… So, in order to fit into the cell, DNA exists in shorter lengths and each length of DNA is tightly wrapped up with histone proteins to form a complex called chromatin. During most of the life of a cell the chromatin is dispersed throughout the nucleus and cannot be seen with a light microscope. However, parts of the chromatin will unwind so that genes on the DNA can be transcribed.
Just before cell division DNA is replicated, and more histone proteins are synthesised, so there
is temporarily twice the normal amount of chromatin. Following replication the chromatin then
coils up even tighter to form short fat bundles called chromosomes.
Chromosomes are about 100,000 times shorter than fully stretched DNA, and therefore 100,000 times thicker, so chromosomes are “large” enough to be seen under a light microscope.
Chromosome have a sort of “X” shape - this is because a chromosome contains two replicated copies of DNA - with each of ‘arms’ of the “X” being identical. Each “arm” of a chromosome is called a chromatid, and chromatids are joined together at the centromere.
DNA molecules extend form one end of a chromosome to the other, and genes are distributed along the DNA. Each gene has its own specific position on a chromosome, and this position is
known as the locus (loci) of the gene.
Some Key terms: -
Chromatin: - DNA + histones at any stage of the cell cycle.
Chromosome: - Compact, short and thick “X” shaped form of chromatin. Chromosomes are condensed forms of chromatin and are therefore visible during mitosis.
Chromatid: - single arm of an “X” shaped chromosome.
A Level Biology - Chromosome Structure and Key Terminology (i.e. Gene, Allele, Locus...)
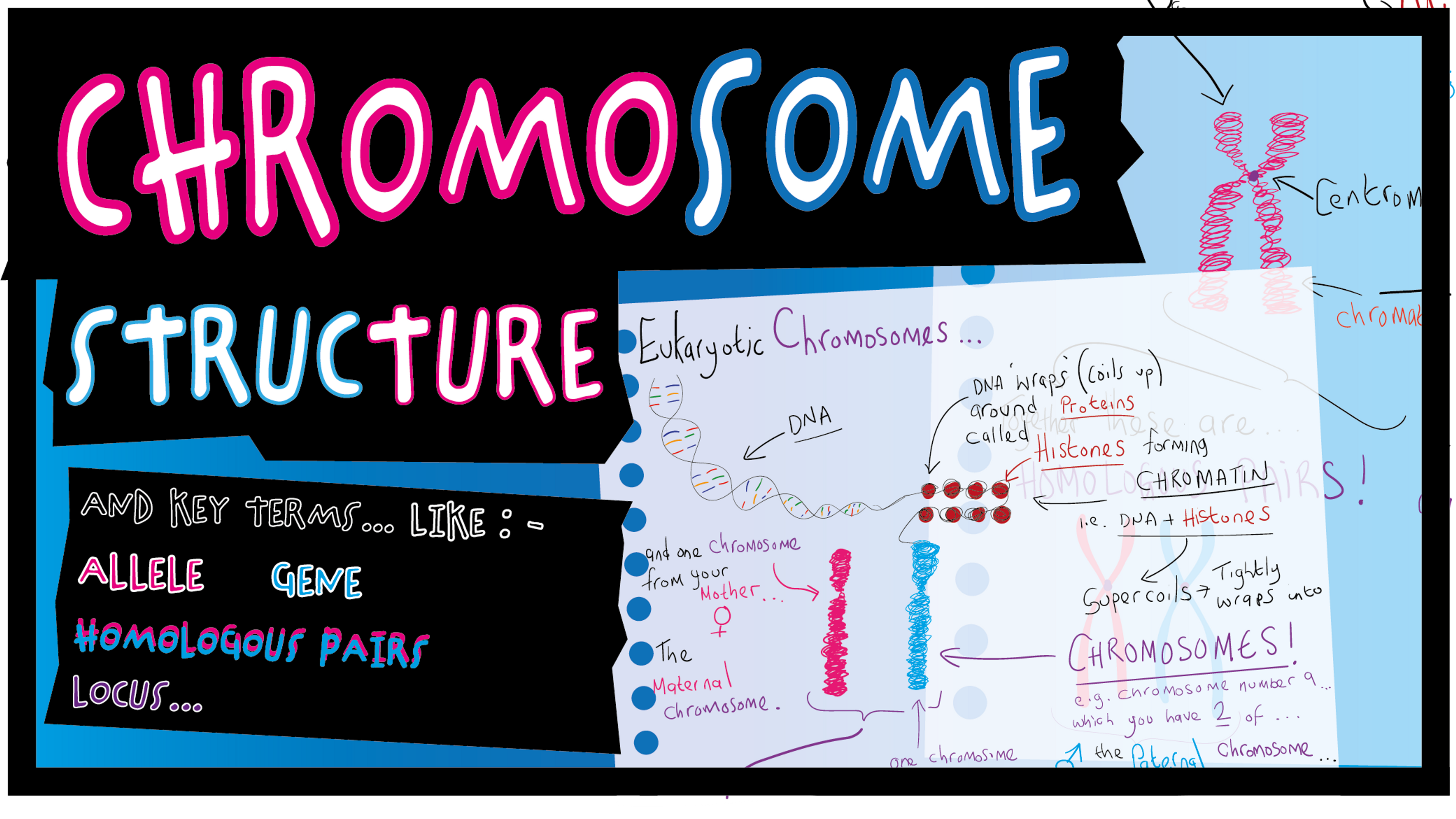
A Level Biology - Genetics:
Defining Diploid - What is meant by 2n?
Most cells in the human body contain 2 sets of chromosomes – they are said to be diploid. The gametes – sperm and ova – are haploid, (they contain only 1 set of chromosomes).
Fertilisation restores the diploid number and the new, genetically unique, organism grows and develops according to the genes it has inherited from both parents.
The term Diploid is standard terminology, expected to be understood and defined for ★ ALL A Level Biology Specifications ★
The term diploid appears in many places in ALL specifications. It’s just a term you must understand and be able to define. Additionally, Students should be able to use the expression 2n to calculate the possible number of different combinations of chromosomes.
A Level Biology - Meiosis
Meiosis as expected to be understood, identified and described by ALL A Level Biology Specifications. Being able to describe Meiosis and identify the stages of meiosis is fundamental for ALL specifications.
Deoxyribonucleic acid (DNA) and Ribonucleic acid (RNA) are perhaps the most important of all biomolecules.
Why?
Well, these nucleic acids contains the instructions that make every single living organism on the planet.
DNA and RNA are polymers, composed of monomers called nucleotides, and it’s now been 67 years since Watson and Crick published their seminal paper proposing the molecular structure of nucleic acids in the prestigious journal ‘Nature’ on the 25th April 1953.
In this section entitled “The structure and function of Nucleic Acids” you’ll learn about the basics of molecular genetics, beginning with the structure and function of nucleotides. From here you’ll be in a much better position to fully understand the structure and function of the most important of all biomolecules - DNA and RNA. Once you have learned all about these structures, you’ll go on to find out about the different types of RNA - in particular messenger RNA (mRNA) and transfer RNA (tRNA), which you must become very familiar since you’ll need to be able to describe the roles of each of these players in protein synthesis. To finish this section you have to learn about genes and non-coding DNA before revisiting how DNA is stored in eukaryotes, prokaryotes and the cellular organelles Mitochondria and Chloroplasts.
In this A-Level Biology Lesson "Nucleic Acids - Nucleotides and Polynucleotides" Following the Learning Outcomes you’ll learn that Nucleotides are the monomers of DNA and RNA. Nucleotides are made up from 3 “parts” - The Pentose Sugar, The Nitrogenous Bases (which can be classified as either Purines or Pyrimidines) and The Phosphate Group. You learn the Structure of a nucleotide and how Condensation Reactions Build Polynucleotides. The lesson will finish with a basic Comparison DNA and RNA.
As you’ll have learned nucleotides have three parts to them: -
1) A pentose sugar (5 carbon atoms in it). By convention the carbon atoms are numbered; 1’, 2', 3’ etc, read as "one prime", “two prime", 3 prime, etc. This convention allows us to distinguish each of the carbon atoms in the base. It is useful when identifying the pentose sugar and describing it to others… for example, if carbon 2' has a hydroxyl group (OH) attached, then the pentose sugar is ribose, found in RNA. However, if carbon 2' just has a hydrogen atom (H) attached, then the sugar is deoxyribose, found in DNA.
2) A phosphate group - which is negatively charged, and gives nucleic acids their acidic properties.
3) A nitrogenous base. There are five different nitrogenous bases (you don't need to remember their molecular structures), but you do need to be aware that of them contain the elements carbon, hydrogen, oxygen and nitrogen, and you’ll also need to know which are Purines and Pyrimidines.
The nitrogenous bases are generally referred to by their first letters only, (but yes, you do need to learn their full names).
There are five nitrogenous bases: -
Adenine (A)
Cytosine (C)
Guanine (G)
Thymine (T) (DNA only)
Uracil (U) (RNA only)
*Note: - The base thymine is only found in DNA and the base uracil is only found in RNA, so there are only four different bases present at a time in one nucleic acid molecule.
DNA contains - A, C, G and T
Whilst RNA contains A, C, G and U
It is also important to note, that informally when talking about nucleotides, we refer to them by the letter name of the nitrogenous base that makes them… for example, the nucleotide ‘A’ (contains the nitrogenous base Adenine - but this nucleotide is actually called “Adenosine” (which you’ll be familiar with from that super imprint molecule ATP (Adenosine triphosphate).
When a nitrogenous base is attached to a Pentose sugar and Phosphate group, the whole molecule is called a nucleotide. So, what are the actual names of the nucleotides: -
When the nitrogen’s base A (Adenine) is attached to a pentose sugar and phosphate group - you already know this nucleotide is called Adenosine.
When C (Cytosine) makes up the nucleotide, the nucleotide is called: Cytidine.
When G (Guanine) makes up the nucleotide, the nucleotide is called: Guanosine.
When T (Thymine) makes up the nucleotide, the nucleotide is called: Thymidine.
and When U (Uracil) makes up the nucleotide, the nucleotide is called: Uridine.
It’s super useful to know this information (since you’ll no doubt read these terms in books, and even exam questions). But you already have a lot to remember and you will not be expected to use these names to answer exam questions.
So why should you be aware of them?
Well learning A-level biology is all about understanding concepts, terminology and being able to apply this knowledge, and when you know the language everything becomes so much easier…
For example: - now you know why ATP is called “Adenosine triphosphate”. Adenosine is the name of the nucleotide and since it has 3 phosphates its called triphosphate.
In fact all nucleotides can have one, two or three phosphate groups. So for instance you can have adenosine monophosphate (AMP), adenosine diphosphate (ADP) and of course adenosine triphosphate (ATP). These nucleotides are very common in cells and have many roles other than just being a component of nucleic acids. ATP as you’ll now by now, is used as an energy storage molecule, while AMP and GTP are used as chemical messengers.
What is Nucleotide Polymerisation?
Polymerisation is biochemical combination of monomers to form polymers, and nucleotides polymerise via condensation reactions between the 3' carbon of the sugar and an oxygen atom of the phosphate group which results in the formation of phosphodiester bonds. The phosphodiester bonds link the pentose sugar to the phosphate - resulting in the sugar-phosphate backbone. Since the nitrogenous bases do not take part in the polymerisation, the sugar-phosphate backbone is made and the nitrogenous bases extending out from it. A polynucleotide has a free phosphate group at one end, this end is called the 5' end (because the phosphate is attached to carbon 5' of the sugar), a free OH group at the other end, called the 3' end (because it's on carbon 3' of the sugar).
The terms 3' and 5' are often used to denote the different ends of a DNA molecule, and will be useful to know when describing how DNA replication take places, or explaining where primers bond during PCR…
Ok, so now you know that nucleotides are super important and you’ve watched the lesson on nucleotides, completed the work booklet and read all this… it’s time to move on to learning about DNA, and a brief history of the key players involved in the “discovery” of this infamous biomolecule.
DNA - The Structure of DNA
DNA is a nucleic acid, (because it is found in the nucleus and is weakly acidic).
DNA is a stable polynucleotide, which means it is composed of many nucleotides and does not begin to denature until it is heated to approximately 86°C. So, DNA is much more stable than proteins! - remember many proteins begin to denature just above 40 °C.
DNA is long molecule and is wound around proteins called histones.
DNA is a polymer: the monomers are nucleotides.
Each nucleotide has three components: -
★ Sugar – deoxyribose – which is a 5-carbon sugar
★ Phosphate group
★ Nitrogenous Base – one of four nitrogen containing compounds: Adenine (A), Thymine (T), Guanine (G) or Cytosine (C).
The nucleotides are arranged in a double helix (a twisted ladder). The two sides of the 'ladder' are chains of alternating sugar–phosphate groups, while the ‘rungs’ of the 'ladder' are made from pairs of bases bonded together by hydrogen bonds.
It is important that, both for protein synthesis and DNA replication, the strands can separate and rejoin without damaging the molecule.
Only one part, of one strand of the DNA molecule, at any particular point in the double-stranded molecule – "the sense strand" – is used to make proteins. The other side serves to stabilise the molecule.
The Nitrogenous bases.
The four bases always bond (via hydrogen bonding) in the same way: –
A hydrogen bonds with T and
C hydrogen bonds to G.
So if you know the base sequence down one side of the DNA molecule, you know the other!
For example, if one strand reads: -
G C G C G G T A C C T A G A T A C A A A A
...then of course the the other (the complementary) side will read: -
C G C G C C A T G G A T C T A T G T T T T
Remember!
The nitrogenous bases are held together by weak hydrogen bonds, you must also remember that there are 2 hydrogen bonds between A and T (i.e. A=T) and 3 hydrogen bonds between C and G (G ≡C).
These regular hydrogen bonds along the whole length of the molecule make DNA very stable.
Chargaff's rules of Complementary Base Pairing.
In 1950 Erwin Chargaff (an American biochemist) concluded that the percentages of DNA bases was equal - following his meeting with Watson and Crick and explaining his findings, the pair used this information to construct their now "infamous" model of showcasing the structure of DNA.
To appreciate the importance of DNA (and genes) in an organism, it is also important to understand the significance of proteins: -
-
Enzymes are proteins that affect the rate of metabolism
-
Structural proteins make up an important part of the fabric of organisms, e.g. collagen, keratin and elastin
-
Antibodies that form a vital part of the immune system are proteins
-
Hormones, e.g. insulin, are proteins
-
Proteins form channels in cell membranes that control what passes in and out of the cell
-
Blood clotting involves many different proteins in a complex sequence of reactions
-
Surface proteins on a cell are unique in each organism, which helps the immune system to differentiate self from non-self and so defend against pathogens / foreign invaders.
Organisms are largely made from proteins, and proteins play many important roles in what happens in our bodies. Yet each individual starts life as a fertilised cell; little more than two sets of genes, made from DNA.
So, When revising A-Level biology be sure to consider the relationship between DNA and Protein
for example: -
The role of nucleic acids in protein and enzyme synthesis.
How the genetic code in DNA is used to build a protein: - i.e. Transcription and Translation.
RNA - The Structure of RNA
The Structure of RNA.
RNA stands for ribonucleic acid, of which there are several types (mRNA, tRNA and rRNA). These different types of RNA are also nucleic acids (like DNA), although RNA is single stranded and not always found in the nucleus...
-
Messenger RNA (mRNA) is a single, long strand of nucleotides that is a copy of a gene (transcription)
-
Transfer RNA (tRNA) is a small, cloverleaf-shaped molecule that brings particular amino acids to the ribosome during translation
-
Ribosomal RNA (rRNA) is a constituent of ribosomes.
mRNA and tRNA
You should be able to construct a table to show the essential differences between DNA and RNA Like this: -
Table to compare DNA and RNA
You have learnt about the structure and functions of DNA and RNA. In this lesson you’ll learn more the types of RNA (specifically mRNA and tRNA) (you should also begin thinking about about how genes are stored in DNA which are used to make proteins).
To make proteins our cells must utilise another nucleic acid - nucleic acid – RNA (RiboNucleic Acid), which exists in 3 different forms: -
-
rRNA
-
mRNA
-
tRNA
RNA is a nucleic acid like DNA, but with 4 key differences: -
-
RNA is made of ribose nucleotides instead of deoxyribose nucleotides
-
RNA has the base uracil instead of thymine
-
RNA is single stranded (though it does fold into 3-dimensional structures)
-
RNA is much shorter than DNA
The are three kinds of RNA, play three different roles: -
Messenger RNA (mRNA)
mRNA - Messenger RNA is responsible for carrying the "message" which codes for a particular sequence of amino acids, and thus the primary sequence of the polypeptide chain. In essence mRNA carries the protein code from DNA in the nucleus (remember DNA carries the “master copy”) to the cytoplasm (where proteins are synthesised).
mRNA is a single stranded molecule and just long enough to contain one gene only (which is approximately 1000 nucleotides in length).
mRNA has a short lifetime and is degraded soon after it is used during the translation of a protein (polypeptide).
Transfer RNA (tRNA)
tRNA - Transfer RNA is an “adapter” that matches amino acids to their codon (on mRNA).
Transfer RNA (tRNA) is only about 80 nucleotides long, and it folds up by complementary base pairing to form a clover-leaf structure which is looped. At one end of the tRNA molecule there is always the base sequence ACC, where the amino acid binds (the amino acid binding site).
On the middle (bottom) loop there is a triplet code - a triplet nucleotide sequence called the anticodon. It is the anticodon which complementary base pairs to a corresponding codon on mRNA during translation.
There are 64 different tRNA molecules, each with a different anticodon sequence complementary to the 64 different codons.
The amino acids are attached to their tRNA molecule by specific aminoacyl tRNA synthase enzymes. These are highly specific, so that each amino acid is attached to a tRNA adapter with the appropriate anticodon.
Ribosomal RNA (rRNA)
rRNA - Ribosomal together with proteins forms ribosomes, which are the sites of
protein synthesis. specifically ribosomes allow mRNA translation to take place, which in turn synthesises proteins. Ribosomes are made up from two subunits, a small subunit and large subunit. Ribosomes are assembled in the nucleolus of the nucleus within cells, and are exported into the cytoplasm. Ribosomal (rRNA) is coded for by numerous genes in many different chromosomes. Ribosomes free in the cytoplasm make proteins for use in the cell, while those attached to the RER make proteins to be exported from the cell.
The Genetic Code
You have previous learnt that the sequence of bases on DNA codes for the sequence of amino acids in proteins (the triplet code).
The sequence is read in groups of three nucleotide bases called codons, where each codon codes for one amino acid.
Each of the 64 codons comprise the genetic code.
[insert The genetic code - mRNA codons / amino acid chart]
Remember the genetic code is degenerate!
-
The code is degenerate, which simply means: “there is often more than one codon for an amino acid”
-
The ‘degeneracy’ is on the third base of the codon, which is therefore less important than the others.
-
One codon means "start" i.e. the start of the gene sequence. It is AUG, which also codes for Methionine (MET) - the “Start Codon”. Therefore, all proteins (primary sequence of a polypeptide chain) start with the amino acid methionine (MET) (although it may be removed later!).
-
When AUG is coded for in the middle of a gene simply codes for the amino acid methionine (i.e its just another amino acid in the sequence - not the “start” signal!)
-
Three codons mean "stop" i.e. the end of the gene sequence. They do not code for amino acids.
-
The code is read from the 5' to 3' end of the mRNA, and the protein is made from the N to C terminus ends.
Genes and non-coding DNA
Genes are sections of DNA (deoxyribonucleic acid) that code for the production of particular proteins.
Humans have about 20,500 genes on our 23 chromosomes. Chromosomes are basically long , coiled DNA molecules.
DNA has two main abilities: -
1) DNA carries genetic information – the genetic code – from which the essential proteins are made.
2) DNA can make exact copies of itself (DNA replication), and it can be copied exactly again and again which means that, when cells divide (see the Cell Cycle and Mitosis), each new cell receives an identical copy of the genetic information in the parent cell.
These abilities make cell division possible, without which there would be no growth or reproduction.
All eukaryotic cells have chromosomes. Each chromosomes is a single, very long molecule of DNA
DNA is highly coiled and folded so that it can fit into the nucleus of a cell.
Remember!
Each gene may come in more than one form. An alternative form of a gene is known as an allele.
For example, the gene that codes for the protein Factor 8 (a vital part of the blood-clotting mechanism) has two alleles: one that codes for the functioning protein and one faulty allele that leads to the disease of haemophilia. Haemophiliacs need injections of Factor 8 to allow their blood to clot normally.
The position of a particular gene on a chromosome is known as its locus. (loci - location of the gene).
Diploid cells have 2 sets of chromosomes
Haploid cells have 1 set.
In diploid cells the chromosomes come homologous pairs; they have the same genes at the same loci
It is important to remember that although homologous chromosomes carry the same genes, they may not carry the same alleles of a gene. If both alleles for a gene are the same (e.g. AA or aa), then the organism is homozygous for that gene. Remember AA = Homozygous Dominant whereas aa = Homozygous recessive. If there are different alleles of a gene on homologous chromosomes (e.g. Aa), then the organism is heterozygous for that gene.
Genetics: Genome, Proteome and Protein Synthesis (summary)
Transcription is when the DNA code on a chromosome is copied into a molecule called messenger RNA (mRNA). The mRNA molecules are effectively mobile copies of genes, since the role of mRNA is to carry the genetic code out of the nucleus and to the site of translation: - the ribosomes in the cytoplasm.
Translation is when the genetic code carried in the mRNA molecule is used to build a polypeptide.
The process of Transcription
Most cells in the human body contain two complete sets of genes. However, only a few genes expressed in any particular cell. For example, every cell in the human body contains two copies of the gene which codes for insulin, but only certain cells in the pancreas actually use the gene to make insulin.
The steps in transcription
-
Step 1. The two strands of DNA unwind along the length of the gene. This event is catalysed by enzymes.
-
Step 2. The enzyme RNA polymerase moves along one side of the DNA molecule – the sense strand containing the genetic code. RNA polymerase catalyses the assembly of a mRNA molecule by the addition of complementary nucleotides. Note. When an RNA molecule is synthesised, the base thymine is replaced by uracil, so the base pairing in RNA is always A with U and C with G.
-
Step 3. The mRNA molecule dissociates from the gene and is now able to move out of the nucleus and on to the site of translation.
Translation
Transfer RNA (tRNA) is the molecule that transfers amino acids to ribosomes during translation. At one end of the tRNA molecule is the anticodon which complementary base pairs to the codon on the mRNA At the other end of the tRNA molecule is the amino acid specified by the codon.
Ribosomes (the sites of protein synthesis) are small dense organelles composed of 2 subunits that hold together all the components needed for translation to take place.
-
Step 1. The mRNA attaches itself to a ribosome.
-
Step 2. The codons are 'translated': e.g. if a codon reads UUU, (for the amino acid phenylalanine), a tRNA molecule with the anticodon AAA will attach, carrying the phenylalanine amino acid at the other end. (Remember the tRNA anticodon is complementary to the mRNA codon).
-
Step 3. Each subsequent codon is translated in the same way, and each successive amino acid is held alongside its neighbour, with peptide bonds which are formed via condensation reactions between each of the amino acids).
This process is repeated: the mRNA molecule moves along the ribosome until a stop codon is encountered, translation stops and the primary sequence of the polypeptide has been constructed.
A-Level Biology: Transcription Exam Style Q and A
What is the Question asking?
It's asking you to describe the process of translation.
What are the key words? Underline the key points in the questions like this: -
What do you know from the Question?
The question tells you what mRNA does and where it is made! (in the Nucleus!) so it is Expected that you can go onto describe in detail the process of Transcription! Its worth 6 marks - so you need a well written answer with 6 key facts about how transcription takes place.
★ THE ANSWER ★
Transcription is the process by which mRNA is made from a DNA template and takes place in the nucleus of a cell. The enzyme helicase breaks hydrogen bonds between complementary base pairs of the DNA helix, exposing the DNA strands. One of the DNA strands acts as a template for the attachment of RNA nucleotides, which also form complementary base pairs (A-U and G-C) joined by the enzyme RNA polymerase. Following elongation of the newly synthesised pre-RNA molecule spicing takes place. An enzyme called a spicersome removes the non-coding regions of the pre-RNA – sections called introns. Whilst the expressed segments are ligated to form the mRNA strand which can now exist the nucleus and is used during translation.
★ NOTE ★
Examiners mark schemes will indicate key words and ideas expected in your answer.
Be aware! Marks can easily be lost if your answer does not include the correct terminology or does not demonstrate an appropriate level of understanding.
Examiners Mark scheme: -
1. Helicase; Breaks hydrogen bonds; 2. Only one DNA strand acts as template; 3. RNA nucleotides attracted to exposed bases.
4. (Attraction) according to base pairing rule; 5. RNA polymerase joins (RNA) nucleotides together;
6. Pre-mRNA spliced to remove introns.
A Level Biology - Genetics: Transcription and RNA Splicing
Transcription - RNA Synthesis
DNA never leaves the nucleus, but proteins are synthesised in the cytoplasm, so a copy of each gene is made to carry the “message” from the nucleus to the cytoplasm. This copy is mRNA, and the process of copying is called transcription.
[image of transcription]
1. The start of each gene on DNA is marked by a special sequence of bases called the promoter.
2. The RNA molecule is built up from the four ribose nucleotides (A, C, G and U) in the nucleoplasm. The
ribose nucleotides attach themselves to the bases on the DNA by complementary base pairing, just as in DNA replication. However, only one strand of RNA is made. The DNA strand that is copied is called
the template strand (or antisense strand). The other strand is a complementary copy, called the nontemplate strand (or sense strand).
3. The new nucleotides are joined to each other by strong covalent phosphodiester bonds by the enzyme RNA polymerase.
4. Only about 8 base pairs remain attached at a time, since the mRNA molecule peels off from the DNA
as it is made. A winding enzyme rewinds the DNA.
5. At the end of the gene the transcription stops, so the mRNA molecule is just the length of the gene.
6. The mRNA diffuses out of the nucleus through a nuclear pore into the cytoplasm. There, it attaches to
ribosomes for translation. It usually doesn't have far to go to find a ribosome, as many are attached to
the rough endoplasmic reticulum, which is contiguous with the nuclear envelope.
Post-Transcriptional Modification (RNA splicing)
Eukaryotic DNA contains non-coding sequences as well as the coding sequences.
The non-coding sequences within genes are called introns, while the coding sequences are called exons.
The introns need to be removed before the mRNA can be translated into protein. This removal is called post-transcriptional modification (or RNA splicing).
[image of splicing]
1. The initial mRNA that is transcribed is called the primary transcript or pre-mRNA. Pre-mRNA is an
exact copy of the gene on the DNA, so it contains exons and introns.
2. The introns in the mRNA are cut out and the exons are joined together by enzymes in a process called
splicing. Some of this splicing is done by the RNA intron itself, acting as an RNA enzyme. The recent
discovery of these RNA enzymes, or ribozymes, illustrates what a diverse and important molecule RNA
is. Other splicing is performed by RNA/protein complexes called snurps.
3. The result is a shorter mature RNA containing only exons. The introns are broken down.
Prokaryotic DNA does not have introns, so mRNA processing is not needed.
Translation - Protein Synthesis
[Image of protein synthesis]
1. A ribosome attaches to the mRNA at an initiation codon (AUG). The ribosome encloses two codons.
2. The first tRNA molecule with an amino acid attached (met-tRNA) diffuses to the ribosome. Its anticodon attaches to the first mRNA codon by complementary base pairing.
3. The next amino acid-tRNA attaches to the adjacent mRNA codon (CUG, leu in this case) by
complementary base pairing.
4. The bond between the amino acid and the tRNA iscut and a peptide bond is formed between the two
amino acids. These operations are catalysed by enzymes in the ribosome called ribozymes.
5. The ribosome moves along one codon so that a new amino acid-tRNA can attach. The free tRNA molecule leaves to collect another amino acid. The cycle repeats from step 3.
6. The polypeptide chain elongates one amino acid at a time, and peels away from the ribosome, folding up into a protein as it goes. This continues for hundreds of amino acids until a stop codon is reached, when the ribosome falls apart, releasing the finished protein.
A single piece of mRNA can be translated by many ribosomes simultaneously, so many protein molecules can be made from one mRNA molecule. A group of ribosomes all attached to one piece of mRNA is called a polyribosome, or a polysome.
[image of polyribosomes (polysome)]
Post-Translational Modification
In eukaryotes, proteins often need to be altered before they become fully functional.
Because this happens after translation, it is called post-translational modification. Modifications are carried out by other enzymes and include: chain cutting, adding methyl or phosphate groups to amino acids, adding sugars (to make glycoproteins) or lipids (to make lipoproteins).
A gene mutation occurs when a change in the sequence of nitrogenous bases occurs, i.e. when a gene is copied incorrectly. This can result in one or more different amino acids being incorporated into the polypeptide (protein). E.g. if a codon reads AAA and is copied incorrectly so that it was read as AAC, then the amino acid argenine would be coded for in place of the amino acid lysine.
There are different types of gene mutation that can occur.
-
Substitution mutation
-
Deletion mutation
-
Addition mutation
A Substitution mutation is where the wrong nitrogenous base is inserted or "substituted" in place of the correct nitrogenous base.
Lets, consider the following nitrogenous base sequence: -
AAT CGG CCC GTA
This will be transcribed into mRNA as: -
UUA GCC GGG CAU
and then translated into the amino acid sequence: -
leucine–alanine–glycine–histidine
However, if just one base is substituted, so that the DNA sequence now reads: -
AAT CAG CCC GTA
The second codon will now be transcribed as GUC which translates into the amino acid valine (replacing the amino acid alanine).
This change to the primary structure of the protein may or may not affect the way the protein functions.
But If the new amino acid caused a significant change to the tertiary and/or quaternary structure (i.e. the final conformational shape of the protein) then the protein may not be able to function properly.
A deletion mutation involves the loss of a nitrogenous base, which results in a frame shift mutation. This can also change most of the codons after the deletion.
Addition mutation is when an extra nitrogenous base is added into the sequence. All the bases that follow in the sequence are moved along (known as a frame shift mutation).
For example: - An addition mutation (where a cytosine bases is added) could cause the following sequence: -
AAT CGG CCC GTA
to become: -
AAC TCG GCC CGT A
This will cause most of the amino acids coded for by codons at or after the addition to change.
Gene mutations can have one of three consequences.
1. The mutation may be lethal. The new amino acid causes the protein to be significantly different resulting in that protein not being able to function properly in the organism. This change could be something like the active site of an enzyme being wrong shape so it cannot combine with its substrate. This could have greater consequences for the organism wherby the enzyme cannot play its part in a sequence of metabolic reactions (like the sequence of events in glycolysis, for example). Mutations that cause frame shifts i.e. additions and deletions, are more likely to be lethal than substitutions because they change more codons, and therefore more amino acids. A protein is far more likely to function properly with just one amino acid change than with a whole new sequence of amino acids.
2. The mutation may have no effect. The protein may still function despite the new amino acid because the change does not alter the tertiary structure or the shape of the part of the protein which interacts with other chemicals. Also, because some amino acid are coded for by several different codons, (Remember the degenerate code) a mutation may not result in a change in the amino acid.
3. The mutation may be beneficial. The new amino acid may alter the protein in such a way that it works in a different way (helping the organism in some way). This will confer a selective advantage on the organism.
The commonly considered example (for A-Level biology and GCSE biology) is the beneficial mutation of the peppered moth (Biston betularia). In the UK before the Industrial Revolution, the 'peppered' (that is the 'speckled') variety of Biston betularia was common.
The 'peppered' markings provided camouflage on lichen covered rocks and trees. However, during Industrial Revolution soot pollution was widespread resulting in the peppered moth being more visible and easily seen and therefore eaten by predators. A random mutation in a gene (that is say a mutation not caused by the soot) resulted in a beneficial mutation that resulted in the production of a black (or melanic) variety of Biston betularia. The mutant moths were better camouflaged on the dark/black sooty surfaces. This gave the melanic variety of Biston betularia a selective advantage. The long term result: The melanic moths became more common in sooty areas than their 'peppered' coloured relatives.
What causes mutations?
Mutations typically occur by chance, that is randomly in the DNA, However, the rate of mutation can be greatly increased by mutagenic agents. sucha as: -
-
ultraviolet light
-
X-rays
-
a and b radiation
-
Chemicals (e.g. mustard gas and cigarette smoke).
Mutations can occur in somatic (body) cells, or in sex cells; sperm or ova.
On the whole though, organisms accumulate somatic cell mutations throughout their lives, as such mutations are not be passed on to their offspring.
Only sex cell (or germ line) mutations are passed on to their offsping.
If the genes that control cell division mutate, a tumour may develop as mitosis takes place in an uncontrolled manner. Tumours (abnormal growth of cells/tissues) may be benign or Malignant.
Gene Mutation
Mutations are changes in genes, which are passed on to daughter cells. DNA is a very stable molecule, and it doesn't suddenly change without reason, but bases can change when DNA is being replicated. Normally replication is extremely accurate, and there are even error-checking procedures in place to ensure accuracy, but very occasionally mistakes do occur (such as a T-C base pair). So a mutation is a base-pairing error during DNA replication.
There are three kinds of gene mutation, shown in this diagram:
[insert image of gene mutations]
Substitution mutations only affect one amino acid, so tend to have less severe effects. In fact if the substitution is on the third base of a codon it may have no effect at all, because the third base often doesn't affect the amino acid coded for (e.g. all codons beginning with CC code for proline). These are called silent mutations. However, if a mutation leads to a premature stop codon the protein will be incomplete and certainly non-functional. This is called a nonsense mutation.
Deletion and insertion mutations have more serious effects because they are frame shift mutations i.e. they change the codon reading frame even though they don't change the actual sequence of bases. So all amino acids "downstream" of the mutation are wrong, and the protein is completely wrong and nonfunctional. However, the effect of a deletion can be cancelled out by a near-by insertion, or by two more deletions, because these will restore the reading frame. A similar argument holds for a substitution.
Mutation Rates and Mutagens
Mutations are normally very rare, which is why members of a species all look alike and can interbreed.
However the rate of mutations is increased by chemicals or by radiation. These are called mutagenic agents
or mutagens, and include:
• High-energy, ionising radiation such as x-rays, ultraviolet rays, a, b, or g rays from radioactive sources.
This ionises the bases so that they don't form the correct base pairs. Note that low-energy radiation
(such as visible light, microwaves and radio waves) doesn't have enough energy to affect DNA and so is harmless.
-
Intercalating chemicals such as mustard gas (used as a weapon in warfare), which bind to DNA separating the two strands.
-
Chemicals that react with the DNA bases such as benzene, nitrous acid, and tar in cigarette smoke.
-
Viruses. Some viruses can change the base sequence in DNA causing genetic disease and cancer.
During the Earth's early history there were far more of these mutagens than there are now, so the mutation rate would have been much higher than now, leading to a greater diversity of life. Some of these mutagens are used today in research, to kill microbes or in warfare. They are often carcinogens since a common result of a mutation is cancer.
Mutations and Cancer
you know that mutations in some genes can cause cancer. Two of these genes are protooncogenes and tumour suppressor genes.
-
Proto-oncogenes encode proteins that act as growth factors by stimulating cell division. The proteins allow cells to pass the checkpoints in the cell cycle and are part of the normal tight control of cell division in organisms. Normally the proto oncogene proteins are only expressed when needed for growth, but if a mutation causes a proto-oncogene to be expressed all the time, then it causes uncontrolled cell division, which can lead to cancer. The mutated gene is then called an ocogene.
-
Tumour-suppressor genes encode proteins that inhibit cell division by blocking the cell cycle checkpoints. Some tumour suppressor genes also initiate cell death (apoptosis). Tumour suppressor genes thus override the effect of oncogenes, and so are also known as anti-oncogenes.
For a cancer to occur, one or more tumour suppressor genes must also be mutated, so their control is removed and cells divide out of control.
[image - metasasis]
So a cancer is usually the result of several different mutations building up.
• A tumour is mass of identical cells (clones) formed by uncontrolled cell division.
-
A benign tumour grows slowly, remains encased in a capsule and does not spread far. Benign tumours are usually harmless (e.g. warts), though they may cause harm by pressing on blood vessels or other tissues. They can often be treated easily by surgery or radiation.
-
A malignant tumour grows quickly and spreads throughout the surrounding tissue, affecting its normal function and so causing harm (e.g. lung cancer reduces elasticity of alveoli). These tumours are more difficult to treat without damaging the whole tissue.
-
A metastasis is a tumour that has spread to the bloodstream or lymphatic system and so can spread to other parts of the body, causing secondary tumours there. These are the most difficult to treat.
Stem Cells
most somatic (body) cells only express a few of their genes, so can only carry out a limited set of functions. These cells are specialised (or differentiated).
In animals specialised cells are irreversibly differentiated, so they and their daughter cells will keep their specialisation and cannot become any other
kind of cell.
However, there are a few cells that remain undifferentiated and so can become any type of cell. These are called stem cells.
Stem cells possess two key properties: -
• Potency – stem cells have the potential to differentiate into specialized cell types.
• Immortality – stem cells can divide indefinitely.
Understanding why stem cells have these properties, but all other cells do not, is a fascinating area of current research. The answers would lead to greater understanding of the process of cell differentiation and the development of cancer.
There are different types of stem cell:-
-
Totipotent (or omnipotent) stem cells can differentiate into any cell type and can even develop into a complete organism. Totipotent stem cells are found in embryos up to the 32-cell stage.
-
Pluripotent stem cells can differentiate into nearly all cells. Pluripotent cells are found in blastocysts (i.e. five-day-old embryos containing around 150 cells, before implantation), so are called embryo stem cells.
-
Multipotent stem cells can differentiate into a related family of cells (e.g. blood cells, muscle cells). Multipotent cells are found in some tissues throughout life, so are called adult stem cells (even though they’re present in children). They are used by the body to replace and repair damaged tissue.
Stem Cells in Medicine
The discovery of stem cells in the 1950s immediately led to suggestions that they could be used clinically.
The idea is to transplant tissue grown from stem cells into a patient, where it would grow and replace damaged tissue. Some potential examples of these cell-based therapies are shown in the table: -
[insert table]
Since the tissues could be grown from the patient’s own stem cells, there is no risk of rejection, unlike conventional transplants. Blood cell-forming (hematopoietic) stem cells from bone marrow are already being used successfully to treat leukaemia (cancer of white blood cells); and heart disease and diabetes have be treated in mice. In addition, human stem cells grown in culture are being used to test the effects of new drugs, without harming humans or animals.
Where do stem cells come from?
-
Embryo stem cells are grown in vitro from human embryos (blastocysts) created by in vitro fertilisation. These embryos are made to help infertile couples reproduce, but any “spare” embryos, no longer needed for reproduction, can be used to create stem cells, with the informed consent of the donor couple. These stem cells are pluripotent, so can be differentiated into a wide variety of different cell types for clinical use. However, there remains a debate about whether it is ethical to use human embryos for this purpose. Embryonic stem cell research had been banned across Europe by the European parliament, although the UK government does allow such research in the UK.
-
Adult stem cells are extracted from certain tissues of the body. It is thought that most organs andtissues contain a small number of undifferentiated stem cells, which the body uses for repair. Tissues where stem cells have been found include the brain, bone marrow, blood vessels, muscle, skin, heart, gut and liver. The use of these cells has no ethical issues, and there are also no problems of rejection if the stem cells are taken from the patient’s own body. However, they are difficult to find and difficult to grow in culture, and they are only multipotent.
-
Induced pluripotent stem cells (iPSCs) are normal, specialised adult cells that have been genetically reprogrammed to become undifferentiated, pluripotent stem cells. iPSCs are a very new development, still at the research stage, but they may solve some of the problems of both adult and embryo stem cells.
Stem Cells in Plants
In plants, unlike animals, some cells remain totipotent throughout life. These cells have the ability to develop into whole plants. These totipotent plant cells are used naturally and artificially: -
• Vegetative Propagation. This is the natural method of asexual reproduction used by plants, where a bud grows from a vegetative (i.e. not reproductive) part of the plant (usually the stem) and develops into a complete new plant, which eventually becomes detached from the parent plant. There are numerous forms of vegetative reproduction, including: bulbs (e.g. onion, daffodil); corms (e.g. crocus, gladiolus); rhizomes (e.g. iris, couch grass); stolons (e.g. blackberry, bramble); runners (e.g. strawberry, creeping buttercup); tubers (e.g. potato, dahlia); tap roots (e.g. carrot, turnip) and tillers (e.g. grasses).
Many of these methods are also perenating organs, which means they contain a food store and are used for survival over winter as well as for asexual reproduction.
Since vegetative reproduction relies entirely on mitosis, all offspring are clones of the parent.
[insert images]
• Cuttings. This is an old artificial method of cloning plants. Parts of a plant stem (or even leaves) are cut off and simply replanted in wet soil. Each cutting produces roots and grows into a complete new plant, so the original plant can be cloned many times. Differentiated cells from tissues in the cut stem start dividing and re differentiating to become root tissue. This division and differentiation is helped if the
cuttings are dipped in “rooting hormone” (a plant growth regulator, such as IAA).
Many flowering plants, such as geraniums, African violet and chrysanthemums are reproduced commercially by cuttings.
[insert images]
-
Tissue Culture (or micropropagation). This is a more modern, and very efficient, way of cloning plants. Small samples of plant tissue, called an explant, can be grown on agar plates in the laboratory in much the same way that bacteria can be grown. Any plant tissue can be used for this (e.g. from a leaf).
The plant tissue can be separated into individual cells, each of which can grow into a mass of undifferentiated cells called a callus. If the correct plant growth regulators (PGRs) are added these cells can develop into whole plantlets, which can eventually be planted outside, where they will grow into normal-sized plants.
Conditions must be kept sterile to prevent infection by microbes.
[insert images]
With micropropagation thousands of clones of a particularly good plant can be made quickly and in a small space. Micropropagation is used on a large scale for fruit trees, ornamental plants and plantation crops such as oil palm, date palm, sugar cane and banana, that cannot be asexually propagated by other means.
Because whole plants can be grown from single cells, this technique could be combined with genetic engineering to grow genetically modified plants from a single genetically-modified cell.
Control of Gene Expression
We’ve seen how genes are expressed through transcription and translation into proteins, which give cells their functions and properties. But cells don’t express all their genes all the time.
Gene expression can be switched on or off by other genes (e.g. during embryo development), by stimuli (e.g. light, injury, nutrients), or by hormones (e.g. growth hormone, oestrogen). There are five control points along the gene expression pathway:
[insert images]
Control of Transcription by Transcription Factors
Transcription is the most important control, since it is the earliest and most efficient point, so mRNA is not made if it’s not needed. We’ve seen that, for transcription to happen, the enzyme RNA polymerase must bind to the DNA molecule just upstream of the gene, at a region called the promoter. However, RNA polymerase binds only weakly at first and it needs the assistance of a number of other DNA-binding proteins, called transcription factors, before it can start transcribing the gene. The transcription factors also bind to the promoter, upstream of the DNA polymerase. DNA is a very flexible molecule and it can loop to allow all the different transcription factors to bind together. This bonding activates RNA polymerase, which can now move along the DNA molecule, transcribing the gene.
[insert images]
This example shows two transcription factors, but in practice there can be over a dozen different proteins involved. Some promote transcription, while others inhibit it, so transcription factors provide a very flexible method of control. Since transcription factors are proteins, they are synthesised in the cytoplasm and transported into the nucleus to bind to DNA. And of course their own production is controlled by
transcription factors!
Steroid hormones control protein synthesis (p22), and they do this via transcription factors. For example oestrogen is secreted by the ovaries and stimulates synthesis of many proteins in target tissues such as the hypothalamus and breasts.
[insert images]
1. Oestrogen, like all steroids hormones, is a lipid, so crosses the cell membrane by lipid diffusion and enters the cytoplasm.
2. Oestrogen binds to its receptor protein (called ERa) in the cytoplasm to form a hormone-receptor complex. This complex is now a transcription factor.
3. The active transcription factor diffuses into the nucleus through a nuclear pore.
4. In the nucleus the transcription factor binds to a specific base sequence on a DNA promoter, upstream of RNA polymerase.
5. This binding stimulates RNA polymerase to transcribe genes and so stimulates protein synthesis.
Control of mRNA by RNA Interference
mRNA molecules have a fairly short lifetime – they are degraded by enzymes after they have been used for translation. The quicker the mRNA is broken down, the less protein can be made, and this is another point where gene expression can be controlled. This control is carried out by special RNA molecules called small interfering RNA (siRNA) and the process is called RNA interference (RNAi).
1. siRNA is a short double-stranded RNA molecule, about 20 base pairs long. It is made by special regulatory genes. These genes are transcribed as normal to make single-stranded RNA, which then folds back on itself by complementary base pairing to make a hairpin-like double-stranded molecule.
2. In the cytoplasm siRNA binds to a protein called the RNA-induced silencing complex (RISC).
3. RISC breaks the double-stranded siRNA into its separate strands. One strand remains attached to the RISC protein, while the other strand is discarded.
[insert images]
4. The RISC-RNA complex now binds to mRNA molecules in the cytoplasm by complementary base pairing. Any mRNA molecules with a base sequence complementary to the 20-base siRNA sequence will bind.
5. This binding causes RISC to cut the mRNA molecule in two.
6. This cleaved mRNA can no longer be used in translation, and is broken down by nuclease enzymes.
RNA interference seems to be mainly used by cells in defence against viral attack. Viruses enter a host cell and use the host cell’s ribosomes to translate viral mRNA and so make more viral proteins. In defence, host cells make siRNA with complementary sequences to viral mRNA, so preventing synthesis of viral proteins.
Applications of RNA interference
Since the discovery of RNA interference in 1998, scientists quickly realised that they could use siRNA to “silence” genes. A 20-base pair siRNA molecule can be synthesised in the laboratory with a sequence that is complementary to part of the gene that is to be silenced. This siRNA is then introduced into cells to observe the effect of silencing that gene.
Applications include: -
-
Identifying the function of genes in cells or simple organisms by silencing the gene and observing the functional effects.
-
Genetically-modifying crop plants by silencing undesirable genes e.g. those that make toxins or allergens. In the Flavr Savr tomato a gene causing ripening was silenced, causing the tomatoes to stay fresh for longer.
-
-
Treating human patients to silence essential genes in cancer cells, or in antiviral therapies e.g. silencing the gene for the receptor protein used by HIV.
Biotechnology
Biotechnology usually refers to the application of molecular (DNA) biology in the laboratory.
Biotechnology has applications in: -
• research e.g. human genome project
• medicine e.g. genetically-engineered drugs, gene therapy
• agriculture e.g. improving crops
• industry e.g. manufacturing enzymes, biosensors
A particular aspect of biotechnology is genetic engineering, which means altering the genes in a living organism to produce a Genetically Modified Organism (GMO) with a new genotype. Genetic engineering can include inserting a foreign gene from one species into another (forming a transgenic organism); altering an existing gene so that its product is changed or changing gene expression.
Techniques of Biotechnology
Modern biotechnology is possible due to the development of techniques from the 1960s onwards, which arose from our greater understanding of DNA and how it functions, following the discovery of its structure by Watson and Crick in 1953. This table lists the techniques that we shall look at in detail.
[insert table of biotech]
-
Restriction Enzymes
These are enzymes that cut DNA at specific sites. They are properly called restriction endonucleases because they cut phosphodiester bonds in the middle of the polynucleotide chain. Some restriction enzymes cut straight across both chains, forming blunt ends, but most enzymes make a staggered cut in the two strands, forming sticky ends.
[insert images]
The cut ends are “sticky” because they have short stretches of single-stranded DNA with complementary sequences. These sticky ends will stick (or anneal) to another sticky end by complementary base pairing (i.e. with weak hydrogen bonds), but only if the sticky ends have both been cut with the same restriction enzyme so that they have complementary sequences. Restriction enzymes have highly specific active sites, and will only cut DNA at specific base sequences, 4-8 base pairs long, called recognition sequences. Recognition sequences are usually palindromic, which means that the sequence and its complement are the same but reversed (e.g. GAATTC has the complement CTTAAG).
Restriction enzymes are produced naturally by bacteria as a defence against viruses (they “restrict” viral growth), but they are enormously useful in genetic engineering for cutting DNA at precise places ("molecular scissors"). Short lengths of DNA cut out by restriction enzymes are called restriction fragments. There are thousands of different restriction enzymes known, with over a hundred different recognition sequences. Restriction enzymes are named after the bacteria species they came from, so EcoR1 is from E. coli strain R, and HindIII is from Haemophilis influenzae.
2. DNA Ligase
This enzyme repairs broken DNA by joining two nucleotides in a DNA strand. Ligase is therefore a bit like DNA polymerase. It is commonly used in genetic engineering to do the reverse of a restriction enzyme, i.e. to join together complementary restriction fragments.
Two restriction fragments can anneal if they have complementary sticky ends, but only by weak hydrogen bonds, which can quite easily be broken, say by gentle heating. The backbone is still incomplete. DNA ligase completes the DNA backbone by forming covalent phosphodiester bonds. Restriction enzymes and DNA ligase can therefore be used together to join lengths of DNA from different sources.
[insert images]
3. Reverse Transcriptase
The enzyme reverse transcriptase does the reverse of transcription: it synthesises DNA from an RNA template (so it is an RNA-dependent DNA polymerase enzyme).
Reverse transcriptase is produced naturally by a group of viruses called the retroviruses (which include HIV), and it helps them to invade cells.
In biotechnology reverse transcriptase is used to make an “artificial gene”, called complementary DNA (cDNA), from an mRNA template as shown in this diagram:
[insert images]
Mature mRNA (without introns) is extracted from cells and mixed with reverse transcriptase and DNA nucleotides. A new strand of DNA is synthesised, complementary to the mRNA strand, forming a doublestranded DNA/RNA “heteroduplex” molecule. The two strands of this molecule are then separated and reverse transcriptase now synthesises a second DNA stand, complementary to the first. The result is a normal double-stranded DNA molecule called cDNA. Note that the cDNA molecule is much shorter than the original gene in the organism’s DNA (typically <50% the size), since the cDNA doesn’t have introns. cDNA is therefore an “artificial gene”.
Reverse transcriptase has several uses in biotechnology:
-
It makes genes without introns. Eukaryotic genes with many introns are often too big to be incorporated into a bacterial plasmid, and bacteria are unable to splice out the introns anyway. The artificial cDNA gene is made from mRNA that already has the introns spliced out of it, so it can be expressed in bacteria.
-
It makes a stable copy of a gene, since DNA is less readily broken down by enzymes than RNA.
-
It makes genes easier to find. There are some 20 000 genes in the human genome, and finding the DNA fragment containing one gene out of this many is a very difficult task. However a given cell only expresses a few genes, so only makes a few different kinds of mRNA molecule. For example the b cells of the pancreas make insulin, so make lots of mRNA molecules coding for insulin. This mRNA can be isolated from these cells and used to make cDNA of the insulin gene.
4. Electrophoresis [link to video]
This is a form of chromatography used to separate different pieces of DNA on the basis of their length. It might typically be used to separate restriction fragments. The DNA samples are placed into wells at one end of a thin slab of gel made of agarose or polyacrylamide, and covered in a buffer solution. An electric current is passed through the gel. Each nucleotide in a molecule of DNA contains a negatively charged phosphate group, so DNA is attracted to the anode (the positive electrode).
The molecules have to diffuse through the gel, and longer lengths of DNA are retarded by the gel so move more slowly than shorter lengths. So the smaller the length of the DNA molecule, the further down the gel it will move in a given time. At the end of the run the current is turned off.
[insert images]
Unfortunately the DNA on the gel cannot be seen, so it must be visualised. There are two common
methods for doing this:
-
The DNA can be stained with a coloured chemical such as azure A (which stains the DNA bands blue), or a fluorescent molecule such as ethidium bromide (which emits coloured light when the finished gel is illuminated with invisible ultraviolet light).
-
The DNA samples at the beginning can be radiolabelled with a radioactive isotope such as 32P, then visualised using autoradiography. Ordinary photographic film (sometimes called X-ray film) is placed on top of the finished gel in the dark for a few hours, and the radiation from any radioactive DNA on the gel exposes the film. When the film is developed the position of the DNA shows up as dark bands on the film. This method is extremely sensitive.
[insert images]
5. DNA Sequencing
This means reading the base sequence of a length of DNA. DNA sequencing is based on a beautifully elegant technique developed by Fred Sanger in Cambridge in 1975, and now called Sanger Sequencing.
-
Label 4 test tubes A, T, C and G. Into each test tube add: a sample of the DNA to be sequenced (containing many millions of individual molecules); a radioactive primer (so the DNA can be visualised later on the gel); the four DNA nucleotides and the enzyme DNA polymerase.
-
In each test tube add a small amount of a special modified dideoxy nucleotide that cannot form a phosphodiester bond and so stops further synthesis of DNA. Tube A has dideoxy A (A*), tube T has dideoxy T (T*), tube C has dideoxy C (C*) and tube G has dideoxy G (G*). The dideoxy nucleotides are present at about 1% of the concentration of the normal nucleotides.
-
Let the DNA polymerase synthesise many copies of the DNA sample. From time to time at random a dideoxy nucleotide will be added to the growing chain and synthesis of that chain will then stop. A range of DNA molecules will be synthesised ranging from full length to very short. The important point is that in tube A, all the fragments will stop at an A nucleotide. In tube T, all the fragments will stop at a T nucleotide, and so on.
-
The contents of the four tubes are now run side by side on an electrophoresis gel, and the DNA bands are visualised by autoradiography. Since the fragments are now sorted by length the sequence can simply be read off the gel starting with the smallest fragment (just one nucleotide) at the bottom and reading upwards.
[insert images]
There is now a modified version of the Sanger method called cycle sequencing, which can be completely automated. The primers are not radiolabelled, but instead the four dideoxy nucleotides are fluorescently labelled, each with a different colour (A* is green, T* is red, C* is blue and G* is yellow). The polymerisation reaction is done in a single tube, using PCR-like cycles to speed up the process. The resulting mixture is separated using capillary electrophoresis, which gives good separation in a single narrow tube gel. The gel is read by a laser beam and the sequence of colours is converted to a DNA sequence by computer program (like the screenshot below). This technique can sequence an amazing 12 000 bases per minute.
Many thousands of genes have been sequenced using these methods and the entire genomes of several
organisms have also been sequenced. A huge project to sequence the complete 3-billion base sequence of the human genome was recently completed. This information is giving us unprecedented knowledge about ourselves, and is likely to lead to dramatic medical and scientific advances. Once a gene sequence is known the amino acid sequence of the protein that the DNA codes for can also be determined, using the genetic code table.
The sequence can also be compared with DNA sequences from other individuals and even other species to work out relationships between individuals or species.
These genome sequences represent vast amount of data that must be analysed and compared to existing sequences. Powerful computers, huge databases and intelligent search programs are being developed to deal with this data, which has led to a whole new branch of biology called bioinformatics, and a new way of doing biology without touching a living thing: in silico.
6. Restriction Mapping
A restriction map is simply a diagram of a piece of DNA marked with the locations of sites where it is cut by restriction enzymes.
A piece of DNA is cut with two different restriction enzymes, both on their own, and together. This gives three different mixtures of restriction fragments, which are run on an electrophoresis gel (labelled E1, E2 and E1+E2 on the gel below). The first lane on this gel contains a “DNA ladder” – a mixture of DNA fragments of known sizes – which is used to calibrate the gel. By comparison with the ladder bands, the length of each restriction fragment can be measured (marked in kilobases, kb on this diagram).
[insert images]
From this information alone we have to deduce the restriction map. Firstly, the fragment lengths in each lane add up to 17, so we know the original DNA was 17kb long. There must be two recognition sites for restriction enzyme 1 (E1), since it gave three bands, while there must be just one recognition site for restriction enzyme 2 (E2), since it gave two bands. By a process of logic, we can construct the following restriction map to account for the banding pattern.
[insert images]
This map is useful in itself, as it can be used to choose restriction enzymes to generate known-sized fragments. But it is even more useful as an aid to DNA sequencing. A long piece of DNA can be cut into shorter restriction fragments, which are easy to sequence. A computer then uses the restriction map to “stitch together” the individual sequences in the correct order and orientation, to generate the complete sequence. The human genome was sequenced in this way.
7. Polymerase Chain Reaction (PCR) [link to video]
The polymerase chain reaction is a technique used to copy (or amplify) DNA samples as small as a single molecule. It was developed in 1985 by Kary Mullis, for which discovery he won a Nobel Prize in 1993. PCR is simply DNA replication in a test tube. If a length of DNA is mixed with the four nucleotides (A, T, C and G) and the enzyme DNA polymerase in a test tube, then the DNA will be replicated many times.
The details are shown in this diagram:
[insert images] [video]
1. Start with a sample of the DNA to be amplified, and add the four nucleotides and the enzyme DNA polymerase.
2. Heat to 95°C for two minutes to breaks the hydrogen bonds between the base pairs and separate the two strands of DNA. Normally (in vivo) the DNA double helix would be separated by an enzyme.
3. Add primers to the mixture and cool to 40°C. Primers are short lengths of single stranded DNA (about 20 bp long) that anneal (i.e. form complementary base pairs) to complementary sequences on the two DNA strands forming short lengths of double-stranded DNA. The DNA is cooled to 40°C to allow the hydrogen bonds to form. There are two reasons for making short lengths of double-stranded DNA:
-
The enzyme DNA polymerase requires some existing double stranded DNA to get it started.
-
Only the DNA between the primer sequences is replicated, so by choosing appropriate primers you can ensure that only a specific target sequence is copied.
4. The DNA polymerase enzyme can now build new stands alongside each old strand to make doublestranded DNA. Each new nucleotide binds to the old strand by complementary base pairing and is joined to the growing chain by a phosphodiester bond. The enzyme used in PCR is derived from the thermophilic bacterium Thermus aquaticus, which grows naturally in hot springs at a temperature of 90°C, so it is not denatured by the high temperatures in step 2. Its optimum temperature is about 72°C, so the mixture is heated to this temperature for a few minutes to allow replication to take place as quickly as possible.
5. Each original DNA molecule has now been replicated to form two molecules. The cycle is repeated from step 2 and each time the number of DNA molecules doubles. This is why it is called a chain reaction, since the number of molecules increases exponentially, like an explosive chain reaction. After n cycles, there is an amplification factor of 2n. Typically PCR is run for 20-30 cycles.
[insert images/video]
PCR can be completely automated, so in a few hours a tiny sample of DNA can be amplified millions of times with little effort. The product can be used for further studies, such as cloning, electrophoresis, or gene probes. Because PCR can use such small samples it can be used in forensic medicine (with DNA taken from samples of blood, hair or semen), and can even be used to copy DNA from mummified human bodies, extinct woolly mammoths, or from an insect that’s been encased in amber since the Jurassic period. One problem of PCR is having a pure enough sample of DNA to start with. Any contaminant DNA will also be amplified, and this can cause problems, for example in court cases.
8. Southern Blot
A Southern blot is used to detect a specific target sequence in samples of DNA. DNA samples separated in an electrophoresis gel can’t be manipulated or stored, since the electrophoresis gel is fragile, and the separated DNA fragments continue to diffuse through it, blurring the bands. So, in a blot, the DNA is transferred to a stronger substrate, where it can be fixed. The target sequence is then detected using a DNA probe.
A DNA probe is simply a short length of single-stranded DNA (100-1000 nucleotides long) with a label attached. There are two common types of label used:
A radioactively-labelled probe (synthesised using the isotope 32P) can be visualised using
autoradiography.
A fluorescently-labelled probe will emit visible light when illuminated with invisible ultraviolet light.
Probes can be made to fluoresce with different colours.
If a probe is added to a mixture of different pieces of single-stranded DNA (e.g. restriction fragments) it will anneal to any fragments of DNA containing the complementary sequence forming regions of doublestranded DNA. This double-stranded DNA is a mixture or hybrid (since it contains DNA from two different sources), so the DNA formed is called hybrid DNA, and the process is called hybridisation. The hybrid DNA fragments will now be labelled and will stand out from the rest of the DNA.
The Southern blot method: -
-
DNA is extracted from the source cells (e.g. different patients or different species) and amplified by PCR to make enough DNA for the hybridisation. The DNA samples are then digested by a restriction enzyme into many small fragments, and the fragments separated on an electrophoresis gel.
-
The gel is placed in an alkali solution, which breaks the hydrogen bonds between the DNA bases causing the stands to separate.
-
A thin sheet of nylon or nitrocellulose is placed on top of the gel and covered with a stack of paper towels, weighted down to make good contact. The alkali solution is drawn up through the gel to the paper towels by capillary action, bringing the DNA with it. The negatively-charged DNA sticks to the positively-charged nylon membrane.
-
After a few hours the DNA fragments are all transferred to the nylon sheet (though of course they can’t be seen). The nylon sheet is separated from the gel, and treated with UV light to fix the DNA molecules.
-
The nylon sheet is placed in a plastic bag containing a solution of labelled probes and mixed thoroughly. The probes will anneal by complementary base-pairing to DNA fragments in the nylon membrane that have a complementary sequence, forming hybrid DNA molecules stuck to the nylon sheet (but again they can’t be seen).
-
The location of the hybrid DNA can be visualised by different methods, depending on the label used. If the probes were radioactive then they can be visualised by autoradiography, and the probes show up as bands on photographic film. If the probes were fluorescent then they can be visualised as bands of light when illuminated by ultraviolet light.
The Southern blot has many uses:
-
To identify restriction fragments containing a particular gene out of the thousands of restriction fragments formed from an entire genome
-
To identify genes in one species that are similar to those of another species.Genes are remarkably similar in sequence from one species to another, so for example a gene probe for a mouse gene will probably anneal with the same gene from a human. This has aided the identification of human genes.
-
To identify the short DNA sequences used in DNA fingerprinting.
-
To screen for genetic diseases, such as Huntington’s disease and cystic fibrosis.
-
The Southern blot was invented by Edwin Southern at Edinburgh University in 1975 (as a biological joke, similar blotting techniques using RNA or protein are called northern and western blots respectively).
9. Genetic Fingerprinting [links to video]
Genetic fingerprinting is used to distinguish between DNA samples from different people. Although 99.9% of human DNA is the same in every person, there is enough variation in the remaining 0.1% (over 100 000 base pairs) to be used to distinguish one individual from another. The differences are found in non-coding
DNA since these regions can accumulate mutations without affecting function, and the differences are largely due to the presence of repetitive sequences. There are different kinds of repetitive sequences: -
Satellite DNA – any DNA region with repetitive sequences. Satellite DNA gets its name because it forms a separate (or satellite) band when separated by density centrifugation.
Tandem repeat – when a simple sequence of bases in a DNA molecule is repeated without a break e.g. ATATATAT (a 2-base repeat) or CCTAAGCCTAAGCCTAAG (a 6-base repeat).
STR (Short Tandem Repeat) or Microsatellite – a tandem repeat with a sequence of less than10 bases.
Minisatellite – a tandem repeat with a sequence of 10–60 bases.
VNTR (Variable Number Tandem Repeat) – a region of DNA containing a particular tandem repeat. While everyone has the same repeating sequence in this region, different individuals have different numbers of repeats. [link to video]
Polymorphism – a range of different phenotypes of a characteristic or genotypes of a gene within a population. In molecular biology it means a range of different DNA sequences at a particular locus.
RFLP (Restriction Fragment Length Polymorphism, pronounced “riff-lip”) – when restriction fragments of the same region of DNA have different lengths in different people.
Genetic Fingerprinting was invented by Sir Alec Jeffreys at Leicester University in 1984. Today there are many different methods of genetic fingerprinting, but Jeffreys’ original method was based on Restriction Fragment Length Polymorphism (RFLPs): -
-
Samples of DNA to be compared (from different individuals) are amplified by PCR. The PCR primers are designed to target and copy a region of the human DNA containing VNTRs.
-
The amplified DNA of the VNTR region is digested by a restriction enzyme. The same recognition sequences should be present in the different samples, so the same number of fragments should be produced, but the fragments will be different lengths depending on the number of tandem repeats in each restriction fragments.
-
The digested DNA is run on an electrophoresis gel to separate out the fragments by size, and the fragments are visualised using a Southern blot. The pattern of bands is different for the two samples because of the restriction fragment length polymorphism i.e. the “same” restriction fragments have different lengths due to different numbers of tandem repeats.
Samples of the same DNA (or from identical twins) give the same banding pattern, but samples from different people give different banding patterns.
[insert images]
This original fingerprinting method takes a few days to carry out and can produce results that are difficult to interpret. Newer methods of genetic fingerprinting have been developed that offer improved speed, sensitivity, discrimination and reliability, but the same principles are used. In the current technique, called DNA profiling and used by the police in most countries of the world, 10-13 different regions containing variable numbers of short tandem repeats (STRs) are targeted and amplified by PCR and then sorted and sized using an automated capillary sequencer. This avoids the need for restriction enzymes or Southern blot and means the process can be automated. The size data can also be stored on computers, forming National DNA Databases. It is always possible that two unrelated people might by chance have the same genetic fingerprint, but using DNA profiling it is calculated that the chance of this is less than 1 in 109.
DNA fingerprinting is used
-
in forensic science, to match DNA samples collected from a crime (e.g. from sperm, blood, hair, skin) with that of suspects. Normally suspects are arrested because of some other link to the crime, but with the development of a national DNA database, that doesn’t have to be the case. Convictions based on DNA evidence can be difficult due to problems of contamination and poor laboratory technique.
-
to determine family relationships, usually between a child and a suspect father (paternity testing). Since children inherit half their DNA from each parent, they inherit half their DNA fingerprint fragments from each parent, so 50% of the bands should match their mother’s bands and 50% match their father’s bands.
-
to prevent undesirable inbreeding during breeding programs in farms and zoos. It can also identify plants or animals that carry a particular desirable (or undesirable) allele.
-
to determine relationships between ancient humans (using DNA extracted from archaeological remains) and modern humans.
-
to establish evolutionary relationships between different species.
-
to measure genetic diversity within a population.
10. Vectors
Now we turn to genetically-modifying living cells. The next three techniques are concerned with transferring genes (DNA) from the test tube into cells, so that the genes can be replicated and expressed in those cells. To do this we first need a vector.
In genetic engineering a vector is a length of DNA that carries the gene we want into a host cell. A vector is needed because a length of DNA containing a gene on its own won’t actually do anything inside a host cell. Since it is not part of the cell’s normal genome it won’t be replicated when the cell divides, it won’t be expressed, and in fact it will probably be broken down pretty quickly. A vector gets round these problems by having these properties:
• It is big enough to hold the gene we want (plus a few others), but not too big.
• It is circular (or more accurately a closed loop), so that it is less likely to be broken down (particularly in prokaryotic cells where DNA is always circular).
• It contains control sequences, such as a replication origin and a transcription promoter, so that the gene will be replicated, expressed, or incorporated into the cell’s normal genome.
• It contains marker genes, so that cells containing the vector can be identified.
Plasmids are the most common kind of vector, so we shall look at how they are used in some detail.
Plasmids are short circular bits of DNA found naturally in bacterial cells. A typical plasmid contains 3-5 genes and there are usually around 10 copies of a plasmid in a bacterial cell. Plasmids are copied separately from the main bacterial DNA when the cell divides, so the plasmid genes are passed on to all daughter cells. They are also used naturally for exchange of genes between bacterial cells, so bacterial cells will readily take up a plasmid.
Because they are so small, plasmids are easy to handle in a test tube, and foreign genes can quite easily be incorporated into them using restriction enzymes and DNA ligase.
One of the most common plasmids used is the R-plasmid. This plasmid contains a replication origin, several recognition sequences for different restriction enzymes (with names like PstI and EcoRI), and two marker genes, which in this case confer resistance to antibiotics. The R plasmid gets its name from these resistance genes.
The diagram below shows how a gene can be incorporated into a plasmid using restriction and ligase
enzymes.
[insert images]
1. A restriction enzyme (Pst1 here) is used to cut the gene from the donor DNA, with sticky ends.
2. The same restriction enzyme cuts the plasmid in the middle of one of the marker genes (we’ll see why this is useful later).
3. The gene and plasmid are mixed in a test tube and they anneal because they were cut with the same restriction enzyme and have the same sticky ends.
4. The fragments are joined covalently by DNA ligase to form a hybrid vector (in other words a mixture or hybrid of bacterial and foreign DNA).
5. Several other products are also formed: some plasmids will simply re-anneal with themselves to re-form the original plasmid, and some DNA fragments will join together to form chains or circles. These different products cannot easily be separated, but it doesn’t matter, as the marker genes can be used later to identify the correct hybrid vector.
Transformation
Transformation means inserting new DNA (usually a plasmid) into a living cell (called a host cell), which is thus genetically modified, or transformed. A transformed cell can replicate and express the genes in the new DNA. DNA is a large molecule that does not readily cross cell membranes, so the membranes must be made permeable in some way. There are different ways of doing this depending on the type of host cell.
• Heat Shock. Bacterial and animal cells in culture can be made to take up DNA from their surroundings by raising the temperature suddenly raised by about 40°C.
-
Electroporation. The most efficient method of delivering genes to bacterial cells is to use a high voltage pulse, which temporarily disrupts the membrane and allows the plasmid to enter the cell.
-
Micro-Injection. To transform individual cells, such as fertilised animal egg cells, the DNA is injected directly into the nucleus using an incredibly fine micro-pipette.
• Gene Gun. Tiny gold particles coated with DNA can be fired at plant cells using a compressed air gun. The particles can penetrate the tough cell wall and deliver the DNA to the nucleus.
• Plant Tumours. Plant cells are infected with a transformed bacterium, which inserts its plasmid into the plant cells' chromosomal DNA. Whole new plants are grown from these cells by micropropagation.
• Liposomes. Human cells in vivo can be transformed by DNA encased in liposomes, which fuse with the cell membrane, delivering the DNA into the cell.
-
Viruses. Human cells in vivo can be infected by genetically-engineered viruses, which deliver the DNA into host cells. The viruses must first be made it safe, so they can’t cause disease.
11. Marker Genes
Marker genes (or reporter genes) are used to find which cells have actually taken up the hybrid vector. Following transformation, there are (at least) these four possible outcomes: -
[insert images]
Vectors contain two different marker genes, which are needed to identify the required cells.
The first marker gene distinguishes between cells that have taken up a plasmid from those that haven’t. The plasmid contains a gene for resistance to an antibiotic such as tetracycline, so bacterial cells taking up this plasmid can make this gene product and so are resistant to this antibiotic. The cells are therefore grown on a medium containing tetracycline, which kills all the normal untransformed cells (>99.99%).
Only the few transformed cells will survive, and these can then be grown and cloned on another plate.
The second marker gene distinguishes between cells that have taken up the hybrid plasmid from those that have taken up the original plasmid. The trick here is that the foreign DNA is inserted inside the second marker gene, so cells with the hybrid plasmid cannot make that gene product. Different genes are used for this second marker: -
-
The marker gene can be a gene for resistance to another antibiotic, such as ampicillin. In this case, cells with the hybrid vector are not resistant to ampicillin.
Since this means killing the cells we want, the ampicillin test is done on a replica plate, where the colonies are transferred to a second agar plate using a cloth stamp. Colonies that grow on the first (tetracycline) plate but not on the replica (ampicillin) plate are the ones we want.
-
The marker gene can be a gene for the enzyme b-galactosidase (lactase). This enzyme turns a white substrate in the agar plate into a blue product. So colonies of cells with the original plasmid turn blue, while those with the hybrid plasmid remain white, and can easily be identified.
• The marker gene can be a gene for green fluorescent protein (GFP). Colonies of cells with the original plasmid fluoresce green in UV light, while those with the hybrid plasmid do not fluoresce, and can easily be identified.
Gene Cloning
Gene cloning simply means make multiple copies of a piece of DNA. It is a necessary step in just about any aspect of molecular biotechnology, such as genetic engineering, genome sequencing or genetic fingerprinting. There are two different ways to clone DNA: -
• In vitro gene cloning uses PCR to clone DNA in the test tube.
• In vivo gene cloning uses restriction enzymes, vectors, DNA ligase, transformation of bacterial cells, marker genes and growth cultures to clone DNA inside bacterial cells.
The two techniques have different advantages and disadvantages: -
[insert table comparing in vitro cloning (using PCR) V in vivo cloning (using living cells) ]
Genetically Modified Organisms
We have looked at some of the many techniques used in biotechnology. We’ll now turn to some applications of these techniques. The applications involve altering the genes in a living organism to produce a Genetically Modified Organism (GMO) with a new genotype. If a foreign gene in copied from one species into another the GMO is called a transgenic organism, but remember that not all GMOs are transgenic: the genetic modification might just alter an existing gene so that its product is changed or change its gene expression. We’ll consider the applications in three groups.
-
Gene Products Using genetically modified organisms (usually microbes) to produce chemicals (usually proteins) for medical or industrial applications.
-
New Phenotypes Using gene technology to alter the characteristics of organisms (usually farm animals or crops).
-
Gene Therapy Using gene technology on humans to treat a disease.
Gene Products.The biggest and most successful kind of genetic engineering is the production of gene products. These products are of medical, agricultural or commercial value to humans. This table shows a few of the examples of genetically engineered products that are already available.
[insert table]
The products are mostly proteins, which are produced directly when a gene is expressed, but they can also be non-protein products produced by genetically engineered enzymes. The basic idea is to transfer a gene (often human) to another host organism (usually a microbe) so that it will make the gene product quickly, cheaply and ethically. It is also possible to make “designer proteins” by altering gene sequences, but while this is a useful research tool, there are no commercial applications yet.
Since the end-product is just a chemical, in principle any kind of organism could be used to produce it. By far the most common group of host organisms used to make gene products are the bacteria, since they can be grown quickly and the product can be purified from their cells. Unfortunately bacteria cannot always make human proteins, and so fungi, animals and plants have also been used to make gene products. This table shows some of the advantages and disadvantages of using different organisms for the production of genetically-engineered gene products.
[insert table Ad V DisAd]
New Phenotypes
This means altering the characteristics of organisms by genetic engineering. The organisms are generally commercially-important crops or farm animals, and the object is to improve their quality in some way. This can be seen as a high-tech version of selective breeding, which has been used by humans to alter and improve their crops and animals for at least 10 000 years. This table gives an idea of what is being done.
[insert table - organisms and their modifications]
Evaluating Biotechnology
The whole point of creating genetically-modified organisms is to benefit humans, and the benefits are usually fairly obvious, but nevertheless there has been some vocal opposition to GMOs. Opposition is often based on ethical, moral or social grounds, such as harm to animals or the environment, though there can also be more practical issues, such as distrust of large corporations.
Benefits
-
Medicines and drugs can be produced safely in large quantities from microbes rather than from slaughtered animals. These medicines benefit humans and can spare animal suffering as well.
-
Agricultural productivity can be improved while using less pesticides or fertilisers, so helping the environment. GM crops can grow on previously unsuitable soil or in previously unsuitable climates.
-
GM crops can improve the nutrition and health of millions of people by improving the nutritional quality of their staple crops.
Risks
• Risks to the modified organism. Genetic modification of an organism may have unforeseen genetic effects on that organism and its offspring. These genetic effects could include metabolic diseases or cancer, and would be particularly important in vertebrate animals, which have a nervous system and so are capable of suffering.
The research process may also harm animals.
-
Transfer to other organisms. Genes transferred into GMOs could be transferred again into other organisms, by natural accidents. These natural accidents could include horizontal gene transmission in bacteria, cross-species pollination in plants, and viral transfer. This could result in a weed being resistant to a herbicide, or a pathogenic bacterium being resistant to an antibiotic. To avoid transfer via crosspollination, genes can now be inserted into chloroplast DNA, which is not found in pollen.
-
Risks to the ecosystems. A GMO may have an unforeseen effect on its food web, affecting other organisms. Many ecosystems are often delicately balanced, and a GMO could change that balance.
-
Risk to biodiversity. GMOs may continue to reduce the genetic biodiversity already occurring due to selective breeding.
-
Risks to human societies. There could be unexpected and complicated social and economic consequences from using GMOs. For example if GM bananas could be grown in temperate countries, that would be disastrous for the economies of those Caribbean countries who rely on banana exports.
-
Risks to local farmers. Developing GMOs is expensive, and the ownership of the technology remains with the large multi-national corporations. This means the benefits may not be available to farmers in third world countries who need it most.
Gene Therapy
The idea of gene therapy is to genetically alter humans in order to treat a disease.
This could represent the first opportunity to cure hitherto incurable diseases. Note that this is quite different from using geneticallyengineered microbes to produce drug, vaccine or hormone to treat a disease by conventional means.
Gene therapy means altering the genotype of a tissue or even a whole human. The most promising targets of gene therapy are single gene disorders (“genetic diseases”) like Huntington’s disease, cystic fibrosis and muscular dystrophy, since these could in principle be treated by replacing just a single gene.
Methods of Gene Therapy
The correct gene (the therapeutic DNA) needs to be introduced into human cells, where it can be expressed. Some of the most common methods are:
• Liposomes. The therapeutic DNA is encased in a lipid vesicle called a liposome. The liposome membrane then fuses with the cell membrane, delivering the therapeutic DNA into the cell.
• Viruses. Normal viral infection depends on the virus delivering its own DNA into host cells, where it can be expressed to make new virus particles. So genetically modified viruses can be used to deliver human genes by the same method. The virus must first be genetically engineered to make it safe, so that it can’t reproduce itself or make toxins.
• Stem cells. In some cases tem cells can be removed from the patient (e.g. from bone marrow), genetically modified in vitro with the therapeutic DNA, then the stem cells injected back into the patient.
This method is safer and avoids immune rejection, but only works for some tissues. Note that gene therapy doesn't alter or replace the existing mutated gene, which will still continue to make the non-functional protein. But in addition, the new gene will make working protein, which will allow the modified cells to function normally.
Problems of Gene Therapy
• Most gene therapy attempted so far has had only a short-lived effect. Problems with integrating the therapeutic DNA into the host cell, and of replicating new DNA when the host cell divides, have meant that patients have to repeat the gene therapy treatment at intervals.
• Therapeutic DNA and modified host cells are recognised as non-self by the immune system and so destroyed in a primary immune response. Subsequent repeated treatments stimulate a greater secondary immune response, which can be harmful to the patient.
• There is a chance that the therapeutic DNA is integrated in the host genome in the middle of another gene, for example in a tumour suppressor gene. The gene therapy could therefore induce cancer. This occurred in a clinical trial of SCID, when 3 out of 20 patients developed leukaemia.
• Viruses are the most successful vectors in gene therapy but they also present a variety of potential problems to the patient including toxicity, immune and inflammatory responses and recovery of pathogenicity. In 1999 a patient in a gene therapy trial died from a massive immune response to the virus being used for gene therapy.
• Many common genetic disorders, such as heart disease, high blood pressure, Alzheimer's disease, arthritis and diabetes, are caused by the combined effects of many genes. These multigene disorders are probably impossible to treat effectively using gene therapy.
Types of Gene Therapy
It is important to appreciate the different between somatic cell therapy and germ line therapy.
• Somatic cell therapy means genetically altering specific body (or somatic) cells, such as trachea epithelial cells, bone marrow cells, pancreas cells, or whatever, in order to treat the disease. This therapy may treat the disease in the patient, but any genetic changes will not be passed on the offspring of the patient.
• Germ-line therapy means genetically altering those cells (sperm cells, sperm precursor cells, ova, ova precursor cells, zygotes or early embryos) that will pass their genes down the “germ-line” to future generations. Alterations to any of these cells will affect every cell in the resulting human, and in all his or her descendants.
Germ-line therapy would be highly effective, but is also potentially dangerous (since the long-term effects of genetic alterations are not known), unethical (since it could easily lead to eugenics) and immoral (since it could involve altering and destroying human embryos). It is currently illegal in the UK and most other countries, and current research is focussing on somatic cell therapy only. All gene therapy trials in the UK must be approved by the Gene Therapy Advisory Committee (GTAC), a government body that reviews the medical and ethical grounds for a trial. Germ-line modification is allowed with animals, and indeed is the basis for producing GMOs.
Genetic Screening and Counselling
There are around 25 000 genes in the human genome, and scientists have now identified and sequenced thousands of human genes. In many cases they also know exactly how the base sequence is different in the different versions (or alleles) of the genes that cause genetic diseases, susceptibility to disease or other clinically-important symptoms. This knowledge can be used to test, or screen, someone for the presence of these clinically-important alleles. The information gained from a screen can be used to select appropriate treatment at an early stage, even before symptoms appear. Even if a genetic disease is incurable, the screen may help in family planning and care of the patient.
Genetic screening is based on DNA hybridisation, and is similar to the Southern blot technique. Short lengths of DNA, 100-1000 nucleotides long, are synthesised with base sequences found only in the mutant alleles. These short lengths of DNA are called gene probes or hybridisation probes. Tiny quantities of these single-stranded gene probes are covalently fixed to a glass or silicon slide in a grid pattern. This slide is called a DNA microarray (or gene chip) and a single microarray a couple of centimetres long can hold 10 000 different gene probes.
The genetic screen procedure is:
1. A few cells are taken from a patient by biopsy.
2. DNA is extracted from the biopsy and if necessary amplified by PCR.
3. The DNA is labelled with a fluorescent chemical.
4. The DNA is denatured (i.e. separated into single strands) by heating or strong alkali.
5. The single-stranded DNA is added to the microarray and mixed for a few hours. DNA that has a complementary sequence to any of the probes fixed to the microarray will hybridise to the probes by complementary base pairing.
6. The microarray is washed with a buffer, washing away any loose fluorescent DNA that is not hybridised to the probes on the chip.
7. If the microarray is illuminated with ultra-violet light, any spots where the subject’s DNA has hybridised to a probe will fluoresce and can be seen, while the spots where the probes haven’t hybridised will remain dark. In practice the microarray is “read” with a laser, which illuminates each spot in turn, recording the amount of fluorescence in each case on a computer.
8. The locations of the fluorescent, “positive”, spots are matched with the name of that probe’s allele, giving a detailed genetic profile of the patient.
Adult screening is carried out to test adults for alleles of late-onset diseases (such as Huntington’s or breast cancer) before any symptoms appear. Adult screening is also used test for carriers of a genetic disease (such as cystic fibrosis). The sample is usually taken by a buccal smear – a scraping of cells from the inside of the cheek.
Newborn screening is carried out immediately after birth, when a blood sample is taken with a lancet from the baby’s heel. DNA is extracted from the baby’s cells and tested for alleles for conditions such as phenylketonuria and congenital hypothyroidism. These conditions benefit from being treated early in life.
Prenatal screening is carried out on fetal cells before birth. It is offered to parents when there is a risk that the fetus might have a serious genetic disability. Amniocentesis is used to collect fetal skin cells in the amniotic fluid between 14 to 20 weeks of gestation, and the DNA from these cells is tested for alleles for conditions such as Tay Sachs disease, sickle cell anaemia, thalassemia, cystic fibrosis, and fragile x syndrome. If the tests are positive the parents have the option of terminating the pregnancy or preparing themselves to care for a disabled child.
Pre-implantation screening (or preimplantation genetic diagnosis, PGD) is performed on human embryos created by in vitro fertilization. One or two cells are carefully removed from an 8-cell embryo and the DNA is tested. This procedure doesn’t harm the rest of the embryo, which continues to develop normally. Only embryos without genetic problems are used for implantation, so this avoids the need for terminations associated with prenatal screening.
Genetic Counselling
The results of genetic screening can be complex and distressing, both for subjects and their families. So genetic screening is often accompanied by genetic counselling. A genetic counsellor can explain the meaning of the test results, discuss the implications for the patient and their family, and advise on the next course of action. For example:
Sickle-cell anaemia is single-gene disorder, caused by a recessive mutation the haemoglobin gene. A healthy woman with a history of sickle-cell anaemia in the family may be a carrier (i.e. heterozygous for the sickle-cell allele). This won’t affect her, but there is a 25% risk that her children may suffer from sickle-cell anaemia if her partner is also a carrier. So before starting a family a couple
with a family history of sickle-cell anaemia may decide to be tested for the sickle cell allele to see if they are both carriers. If they are the genetic counsellor could discuss the options of pre-natal or preimplantation screening.
Huntington’s disease is a single-gene disorder caused by a dominant mutation in the huntingtin gene. Huntington’s disease causes muscle spasms and death, but there are no symptoms until middle age, and there is no cure. Someone with a history of Huntington’s disease in the family may choose to be tested for the mutated allele. Since this is a dominant allele, a positive test result means that they will develop the disease in their forties, and there is a 50% chance that they will pass the disease on to any children they may have. The test can also indicate the likely severity of the disease. Genetic counselling and support is very important in this case since the impact on the individual and their family are so severe. 95% of individuals at risk choose not to under genetic testing, since there is no cure. The uncertainly of not knowing whether they will develop Huntington’s disease must be weighed against the stress of knowing that they eventually will, or even the “survivor guilt” of knowing that they don’t have the disease.
Cancers can be caused by mutations in many different genes, such as oncogenes and tumour suppressor genes. Gene probes have been made for hundreds of these cancer-related alleles, so they can be tested in genetic screens. Screening and counselling can help to choose the best treatment for a particular patient. For example the drug herceptin is only effective at treating a certain type of breast cancer, caused by mutation in the HER2 gene.
There can be other issues with genetic screening. Mistakes in procedures or interpretation can result in false-positive or false-negative results. Results of genetic tests must remain confidential since they may reveal information about the subject’s family and their future health prospects. This could lead to genetic discrimination, where people are denied jobs or insurance because of their genes. In the USA it is illegal for a company to discriminate for employment or health insurance on the basis of genetic test results.
Recombinant DNA (and the central dogma of biology)
Making cDNA with using the enzyme Reverse Transcriptase
A Level Biology Gene Technologies: Making cDNA using the Enzyme Reverse Transcriptase.
In this lesson we will focus on how DNA clones called cDNA can be produced using reverse transcriptase.
Remember that retroviruses (like HIV) have the enzyme reverse transcriptase.
mRNA is required to direct the synthesis of a polypeptide chain
Using Reverse transcriptase - Step 1.
Using Reverse transcriptase - Step 2.
Using Reverse transcriptase - Step 3.
Using Reverse transcriptase - Step 4.
Using Reverse transcriptase - Step 5.
the cDNA can be inserted into viral vectors or plasmid.
Exam Style Q and A (1)
Exam Style Q and A (2)
Exam Style Q and A (3)
Reverse Transcriptase
The enzyme reverse transcriptase does the reverse of transcription: it synthesises DNA from an RNA template (so it is an RNA-dependent DNA polymerase enzyme). Reverse transcriptase is produced naturally by a group of viruses called the retroviruses (which include HIV), and it helps them to invade cells.
In biotechnology reverse transcriptase is used to make an “artificial gene”, called complementary DNA (cDNA), from an mRNA template.
Mature mRNA (without introns) is extracted from cells and mixed with reverse transcriptase and DNA nucleotides. A new strand of DNA is synthesised, complementary to the mRNA strand, forming a double-stranded DNA/RNA “heteroduplex” molecule. The two strands of this molecule are then separated and reverse transcriptase now synthesises a second DNA strand, complementary to the first. The result is a normal double-stranded DNA molecule called cDNA. Note that the cDNA molecule is much shorter than the original gene in the organism’s DNA (typically <50% the size), since the cDNA doesn’t have introns. cDNA is therefore an “artificial gene”.
Reverse transcriptase has several uses in biotechnology:
-
It makes genes without introns. Eukaryotic genes with many introns are often too big to be incorporated into a bacterial plasmid, and bacteria are unable to splice out the introns anyway. The artificial cDNA gene is made from mRNA that already has the introns spliced out of it, so it can be expressed in bacteria.
-
It makes a stable copy of a gene, since DNA is less readily broken down by enzymes than RNA.
-
It makes genes easier to find. There are some 20 000 genes in the human genome, and finding the DNA fragment containing one gene out of this many is a very difficult task. However a given cell only expresses a few genes, so only makes a few different kinds of mRNA molecule. For example the b cells of the pancreas make insulin, so make lots of mRNA molecules coding for insulin. This mRNA can be isolated from these cells and used to make cDNA of the insulin gene.
PCR - The Polymerase Chain Reaction
A Level Biology the polymerase chain reaction (PCR)
Introduction | Learning Outcomes
The Polymerase Chain Reaction
Why is PCR called PCR? / 1. "Polymerase"
"Chain Reaction"
"In Vitro"
What are Thermostable DNA Polymerase Enzymes?
"1985" Kary Mullis
Primers and annealing
What you need to know for your A level exams...
PCR - Step 1. Denaturation
PCR - Step 2. Annealing
PCR - Step 3. Polymerisation
PCR Amplifies DNA Exponentially with each cycle.
Applications of PCR (environmental, medical and forensic).
Polymerase Chain Reaction (PCR)
The polymerase chain reaction is a technique used to copy (or amplify) DNA samples as small as a single molecule. It was developed in 1985 by Kary Mullis, for which discovery he won a Nobel Prize in 1993. PCR is simply DNA replication in a test tube. If a length of DNA is mixed with the four nucleotides (A, T, C and G) and the enzyme DNA polymerase in a test tube, then the DNA will be replicated many times.
1. Start with a sample of the DNA to be amplified, and add the four nucleotides and the enzyme DNA polymerase.
2. Heat to 95°C for two minutes to breaks the hydrogen bonds between the base pairs and separate the two strands of DNA. Normally (in vivo) the DNA double helix would be separated by an enzyme.
3. Add primers to the mixture and cool to 40°C. Primers are short lengths of single stranded DNA (about 20 bp long) that anneal (i.e. form complementary base pairs) to complementary sequences on the two DNA strands forming short lengths of double-stranded DNA. The DNA is cooled to 40°C to allow the hydrogen bonds to form. There are two reasons for making short lengths of double-stranded DNA:
-
The enzyme DNA polymerase requires some existing double stranded DNA to get it started.
-
Only the DNA between the primer sequences is replicated, so by choosing appropriate primers you can ensure that only a specific target sequence is copied.
4. The DNA polymerase enzyme can now build new stands alongside each old strand to make double-stranded DNA. Each new nucleotide binds to the old strand by complementary base pairing and is joined to the growing chain by a phosphodiester bond. The enzyme used in PCR is derived from the thermophilic bacterium Thermus aquaticus, which grows naturally in hot springs at a temperature of 90°C, so it is not denatured by the high temperatures in step 2. Its optimum temperature is about 72°C, so the mixture is heated to this temperature for a few minutes to allow replication to take place as quickly as possible.
5. Each original DNA molecule has now been replicated to form two molecules. The cycle is repeated from step 2 and each time the number of DNA molecules doubles. This is why it is called a chain reaction, since the number of molecules increases exponentially, like an explosive chain reaction. After n cycles, there is an amplification factor of 2n. Typically PCR is run for 20-30 cycles.
PCR can be completely automated, so in a few hours a tiny sample of DNA can be amplified millions of times with little effort. The product can be used for further studies, such as cloning, electrophoresis, or gene probes. Because PCR can use such small samples it can be used in forensic medicine (with DNA taken from samples of blood, hair or semen), and can even be used to copy DNA from mummified human bodies, extinct woolly mammoths, or from an insect that’s been encased in amber since the Jurassic period. One problem of PCR is having a pure enough sample of DNA to start with. Any contaminant DNA will also be amplified, and this can cause problems, for example in court cases.
Gene Technologies DNA Profiling | Genetic Fingerprinting using (VNTRs)
Introduction | Learning Outcomes.
Genomes.
VNTR a.k.a Mini-satellite sequences and restriction Fragment Length Polymorphisms (RFLP) Remember - Chromosomes are homologous...
How VNTRs are useful...
Electrophoresis / Paternity Testing.
1 in 10 billion!
DNA Fingerprinting uses PCR and VNTRs...
Using VNTRs and Electrophoresis in Forensic Science...
The "suspects" DNA profiles are Unique.
PCR and VNTRs have many uses...
Calculating the number of nucleotides in a VNTR.
Genetic Fingerprinting
Genetic fingerprinting is used to distinguish between DNA samples from different people. Although 99.9% of human DNA is the same in every person, there is enough variation in the remaining 0.1% (over 100 000 base pairs) to be used to distinguish one individual from another. The differences are found in non-coding
DNA since these regions can accumulate mutations without affecting function, and the differences are largely due to the presence of repetitive sequences. There are different kinds of repetitive sequences: -
Satellite DNA – any DNA region with repetitive sequences. Satellite DNA gets its name because it forms a separate (or satellite) band when separated by density centrifugation.
Tandem repeat – when a simple sequence of bases in a DNA molecule is repeated without a break e.g. ATATATAT (a 2-base repeat) or CCTAAGCCTAAGCCTAAG (a 6-base repeat).
STR (Short Tandem Repeat) or Microsatellite – a tandem repeat with a sequence of less than10 bases.
Minisatellite – a tandem repeat with a sequence of 10–60 bases.
VNTR (Variable Number Tandem Repeat) – a region of DNA containing a particular tandem repeat. While everyone has the same repeating sequence in this region, different individuals have different numbers of repeats. [link to video]
Polymorphism – a range of different phenotypes of a characteristic or genotypes of a gene within a population. In molecular biology it means a range of different DNA sequences at a particular locus.
RFLP (Restriction Fragment Length Polymorphism, pronounced “riff-lip”) – when restriction fragments of the same region of DNA have different lengths in different people.
Genetic Fingerprinting was invented by Sir Alec Jeffreys at Leicester University in 1984. Today there are many different methods of genetic fingerprinting, but Jeffreys’ original method was based on Restriction Fragment Length Polymorphism (RFLPs): -
-
Samples of DNA to be compared (from different individuals) are amplified by PCR. The PCR primers are designed to target and copy a region of the human DNA containing VNTRs.
-
The amplified DNA of the VNTR region is digested by a restriction enzyme. The same recognition sequences should be present in the different samples, so the same number of fragments should be produced, but the fragments will be different lengths depending on the number of tandem repeats in each restriction fragments.
-
The digested DNA is run on an electrophoresis gel to separate out the fragments by size, and the fragments are visualised using a Southern blot. The pattern of bands is different for the two samples because of the restriction fragment length polymorphism i.e. the “same” restriction fragments have different lengths due to different numbers of tandem repeats.
Samples of the same DNA (or from identical twins) give the same banding pattern, but samples from different people give different banding patterns.
This original fingerprinting method takes a few days to carry out and can produce results that are difficult to interpret. Newer methods of genetic fingerprinting have been developed that offer improved speed, sensitivity, discrimination and reliability, but the same principles are used. In the current technique, called DNA profiling and used by the police in most countries of the world, 10-13 different regions containing variable numbers of short tandem repeats (STRs) are targeted and amplified by PCR and then sorted and sized using an automated capillary sequencer. This avoids the need for restriction enzymes or Southern blot and means the process can be automated. The size data can also be stored on computers, forming National DNA Databases. It is always possible that two unrelated people might by chance have the same genetic fingerprint, but using DNA profiling it is calculated that the chance of this is less than 1 in 109.
DNA fingerprinting is used
-
in forensic science, to match DNA samples collected from a crime (e.g. from sperm, blood, hair, skin) with that of suspects. Normally suspects are arrested because of some other link to the crime, but with the development of a national DNA database, that doesn’t have to be the case. Convictions based on DNA evidence can be difficult due to problems of contamination and poor laboratory technique.
-
to determine family relationships, usually between a child and a suspect father (paternity testing). Since children inherit half their DNA from each parent, they inherit half their DNA fingerprint fragments from each parent, so 50% of the bands should match their mother’s bands and 50% match their father’s bands.
-
to prevent undesirable inbreeding during breeding programs in farms and zoos. It can also identify plants or animals that carry a particular desirable (or undesirable) allele.
-
to determine relationships between ancient humans (using DNA extracted from archaeological remains) and modern humans.
-
to establish evolutionary relationships between different species.
-
to measure genetic diversity within a population.
Gel Electrophoresis
Gel Electrophoresis
This is a form of chromatography used to separate different pieces of DNA on the basis of their length. It might typically be used to separate restriction fragments. The DNA samples are placed into wells at one end of a thin slab of gel made of agarose or polyacrylamide, and covered in a buffer solution. An electric current is passed through the gel. Each nucleotide in a molecule of DNA contains a negatively charged phosphate group, so DNA is attracted to the anode (the positive electrode).
The molecules have to diffuse through the gel, and longer lengths of DNA are retarded by the gel so move more slowly than shorter lengths. So the smaller the length of the DNA molecule, the further down the gel it will move in a given time. At the end of the run the current is turned off.
Unfortunately the DNA on the gel cannot be seen, so it must be visualised. There are two common methods for doing this:
-
The DNA can be stained with a coloured chemical such as azure A (which stains the DNA bands blue), or a fluorescent molecule such as ethidium bromide (which emits coloured light when the finished gel is illuminated with invisible ultraviolet light).
-
The DNA samples at the beginning can be radiolabeled with a radioactive isotope such as 32P, then visualised using autoradiography. Ordinary photographic film (sometimes called X-ray film) is placed on top of the finished gel in the dark for a few hours, and the radiation from any radioactive DNA on the gel exposes the film. When the film is developed the position of the DNA shows up as dark bands on the film. This method is extremely sensitive.
A Level Biology - The Hardy-Weinberg Principle
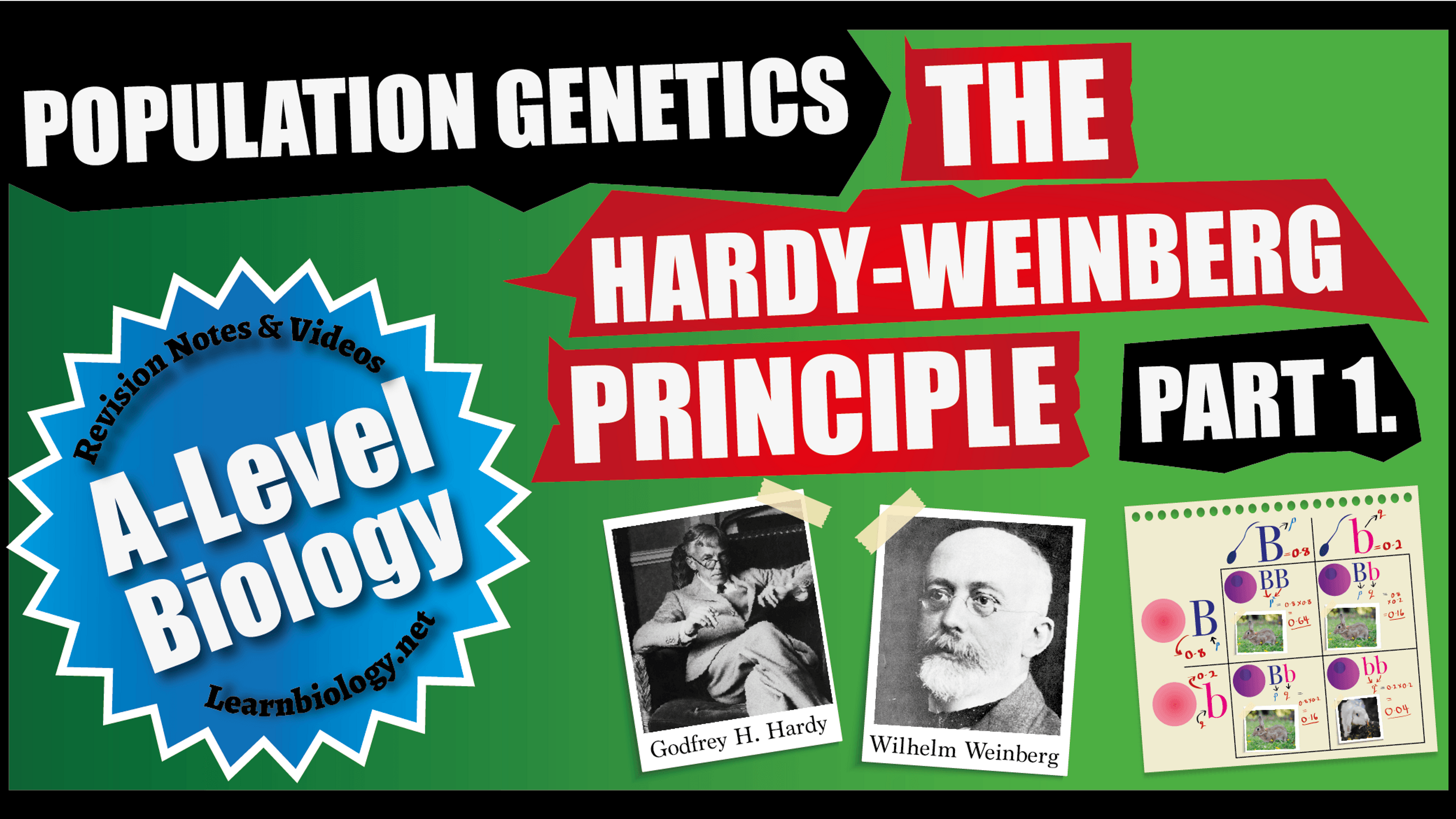
A Level Biology - The Hardy-Weinberg Principle (part 2)
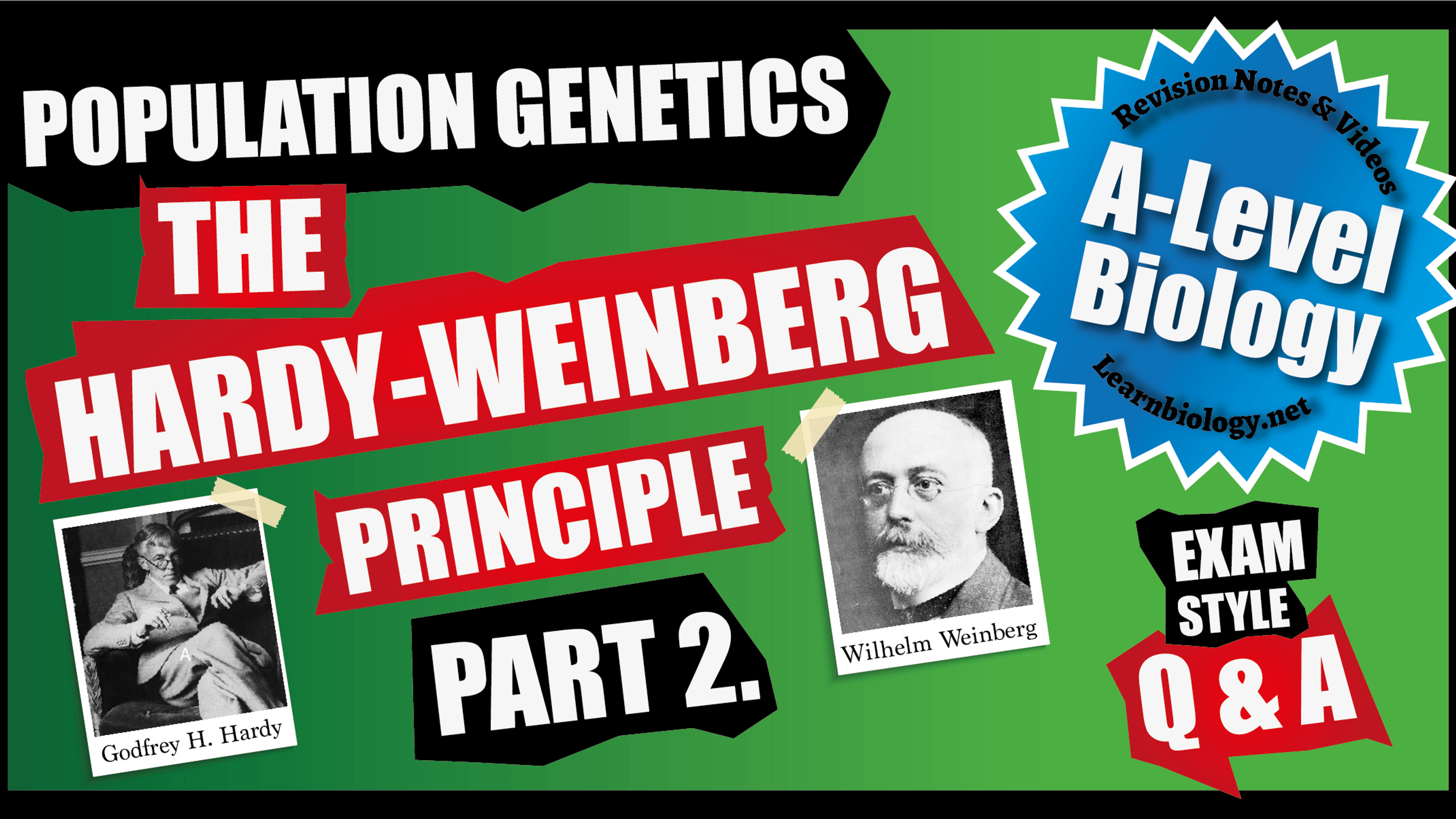
Check Your Exam Specification
★ AQA Specification Reference: - 3.1.5.1 Structure of DNA and RNA. Both DNA and RNA are polymers of nucleotides. Each nucleotide is formed from a pentose, a nitrogen-containing organic base and a phosphate group: -
• The components of a DNA nucleotide are deoxyribose, a phosphate group and one of the organic bases adenine, cytosine, guanine or thymine.
• The components of an RNA nucleotide are ribose, a phosphate group and one of the organic bases adenine, cytosine, guanine or uracil.
• A condensation reaction between two nucleotides forms a phosphodiester bond.
★ CIE Specification Reference: - 6 Nucleic acids and protein synthesis. 6.1 Structure and replication of
DNAa) describe the structure of nucleotides.
★ Edexcel (Biology A – Salters-Nuffield) Specification Reference: - Topic 2: Genes and Health. 2.5 i) Know the basic structure of mononucleotides (deoxyribose or ribose linked to a phosphate and a base, including thymine, uracil, cytosine, adenine or guanine) and the structures of DNA and RNA (polynucleotides composed of mononucleotides linked through condensation reactions).
★ Edexcel (Biology B) Specification Reference: - Topic 1: Biological Molecules. 1.1 Carbohydrates ii) Know the structure of the pentose ribose (and deoxyribose).1.4 DNA and protein synthesis i) Know the structure of DNA, including the structure of the nucleotides (purines and pyrimidines), the sugar-phosphate backbone and phosphodiester bonds.
★ OCR (Biology A) Specification Reference: - Module 2: Foundations in biology –
2.1.2 Biological molecules. (d) the ring structure of ribose as an example of a pentose monosaccharide. 2.1.3 Nucleotides and nucleic acids. (a) the structure of a nucleotide as the monomer from which nucleic acids are made. To include the differences between RNA and DNA nucleotides, the identification of the purines and pyrimidines and the type of pentose sugar. (b) the synthesis and breakdown of polynucleotides by the formation and breakage of phosphodiester bonds.
★ OCR (Biology B) Specification Reference: - 2.1.4 Nucleic acids. (a) the structure of a nucleotide as the monomer from which nucleic acids are made - To include the differences between RNA and DNA nucleotides, the identification of the purines and pyrimidinesthe type of pentose sugar and the formation of phosphodiester bonds (the sugar-phosphate backbone).
★ WJEC Specification Reference: - Basic Biochemistry and Cell Organisation (1). (c) the structure, properties and functions of carbohydrates: pentose sugars Ribose and Deoxyribose).
Basic Biochemistry and Cell Organisation (5). Nucleic acids and their functions, (a) the structure of nucleotides (pentose sugar, phosphate, organic base). (e) the structure of nucleic acids: DNA bases: purines and pyrimidines. (f) the similarities and differences in the structure of RNA and DNA
★ AQA Specification Reference: - 3.1.5 Nucleic acids are important information-carrying molecules. 3.1.5.1 Structure of DNA. Deoxyribonucleic acid (DNA) is an important information-carrying molecules. In all living cells, DNA holds genetic information. DNA is a polymer of nucleotides. Each nucleotide is formed from a pentose, a nitrogen containing organic base and a phosphate group. The components of a DNA nucleotide are deoxyribose, a phosphate group and one of the organic bases adenine, cytosine, guanine or thymine. A condensation reaction between two nucleotides forms a phosphodiester bond. A DNA molecule is a double helix with two polynucleotide chains held together by hydrogen bonds between specific complementary base pairs. Students should be able to appreciate that the relative simplicity of DNA led many scientists to doubt that it carried the genetic code.
★ CIE Specification Reference: - 6 Nucleic acids. 6.1 Structure of DNA. Understanding the structure of nucleic acids allows an understanding of their role in the storage of genetic information. a) describe the structure of nucleotides. b) describe the structure of DNA and explain the importance of base pairing and the different hydrogen bonding between bases (include reference to adenine and guanine as purines and to cytosine, thymine and uracil as pyrimidines. Structural formulae for bases are not required but the recognition that purines have a double ring structure and pyrimidines have a single ring structure should be included).
★ Edexcel (Biology A – Salters-Nuffield) Specification Reference: - Topic 2: Genes and Health. 2.5 i) Know the basic structure of mononucleotides (deoxyribose linked to a phosphate and a base, including thymine, cytosine, adenine or guanine) and the structures of DNA (polynucleotide composed of mononucleotides linked through condensation reactions). ii) Know how complementary base pairing and the hydrogen bonding between two complementary strands are involved in the formation of the DNA double helix.
★ Edexcel (Biology B) Specification Reference: - Topic 1: Biological Molecules. 1.4 DNA and protein synthesis. i) Know the structure of DNA, including the structure of the nucleotides (purines and pyrimidines), base pairing, the two sugar-phosphate backbones, phosphodiester bonds and hydrogen bonds.
★ OCR (Biology A) Specification Reference: - 2.1.3 Nucleotides and nucleic acids. (a) the structure of a nucleotide as the monomer from which nucleic acids are made (the identification of the purines and pyrimidines and the type of pentose sugar and the formation of phosphodiester bonds (the sugar phosphate backbone. (b) the synthesis and breakdown of polynucleotides by the formation and breakage of phosphodiester bonds. (d) (i) the structure of DNA (deoxyribonucleic acid) To include how hydrogen bonding between complementary base pairs (A to T, G to C) on two antiparallel DNA polynucleotides leads to the formation of a DNA molecule, and how the twisting of DNA produces its ‘double-helix’ shape.
★ OCR (Biology B) Specification Reference: - 2.1.4 Nucleic acids. (a) the structure of a nucleotide as the monomer from which nucleic acids are made (the identification of the purines and pyrimidines and the type of pentose sugar and the formation of phosphodiester bonds (the sugar phosphate backbone). (c) (i) the structure of the DNA molecule, including a review of the evidence for complementary bases pairing “Chargaff’s rules”).
★ WJEC Specification Reference: - Core Concepts 5. Nucleic acids and their functions. (a) the structure of nucleotides (pentose sugar, phosphate, organic base). (e) the structure of nucleic acids: DNA bases: purines and pyrimidines; complementary base pair rule; hydrogen bonding and the double helix; antiparallel strands.
★ AQA Specification Reference: - 3.1.5 The Structure of RNA.
★ CIE Specification Reference: - 6 Nucleic acids. 6.1 Structure of RNA. Understanding the structure of nucleic acids allows an understanding of their role in the storage of genetic information.
★ Edexcel (Biology A – Salters-Nuffield) Specification Reference: - Topic 2: Genes and Health. 2.5 i) Know the basic structure RNA
★ Edexcel (Biology B) Specification Reference: - Topic 1: Biological Molecules. Know the structure of RNA.
★ OCR (Biology A) Specification Reference: - 2.1.3 Nucleotides and nucleic acids. (a) the structure of a nucleotide as the monomer from which nucleic acids are made (the identification of the purines and pyrimidines and the type of pentose sugar and the formation of phosphodiester bonds (the sugar phosphate backbone. Know the Structure of RNA
★ OCR (Biology B) Specification Reference: - 2.1.4 Nucleic acids. (a) the structure of a nucleotide as the monomer from which nucleic acids are made (the identification of the purines and pyrimidines and the type of pentose sugar and the formation of phosphodiester bonds (the sugar phosphate backbone). (c) (i) the structure of the RNA molecule.
★ WJEC Specification Reference: - Core Concepts 5. Nucleic acids and their functions. (a) the structure of nucleotides (pentose sugar, phosphate, organic base). (e) the structure of nucleic acids: RNA.
★ AQA A Level Biology Specification Reference: - 3.4.1 DNA, genes and chromosomes: In prokaryotic cells, DNA molecules are short, circular and not associated with proteins. In the nucleus of eukaryotic cells, A gene occupies a fixed position, called a locus, on a particular DNA molecule. A sequence of three DNA bases, called a triplet, codes for a specific amino acid. The genetic code is universal, non-overlapping and degenerate. In eukaryotes, much of the nuclear DNA does not code for polypeptides. There are, for example, non-coding multiple repeats of base sequences between genes. Even within a gene only some sequences, called exons, code for amino acid sequences. Within the gene, these exons are separated by one or more non-coding sequences, called introns.
★ CIE A Level Biology Specification Reference: - 6 Nucleic acids and protein synthesis. Explain the term gene. Describe how the information in DNA is used during transcription and translation to construct polypeptides, including the role of messenger RNA (mRNA), transfer RNA (tRNA) and the ribosomes
★ Edexcel A Level Biology (Biology A – Salters-Nuffield) Specification Reference: - Topic 2: Genes and Health: 2.8 Know that a gene is a sequence of bases on a DNA molecule that codes for a sequence of amino acids in a polypeptide chain.
★ Edexcel A Level Biology (Biology B) Specification Reference: - Know that a gene is a sequence of bases on a DNA molecule coding for a sequence of amino acids in a polypeptide chain.
★ OCR A Level Biology (Biology A) Specification Reference: - 6.1 Genetics and evolution - 6.1.1 Cellular control: the regulatory mechanisms that control gene expression at the transcriptional level, posttranscriptional level and post-translational level - post-transcriptional level: the editing of primary mRNA and the removal of introns to produce mature mRNA.
★ OCR A Level Biology (Biology B) Specification Reference: - 5.1.3 Gene technologies - post transcriptional editing of mRNA. To include the production of mature mRNA in human cells, the nature of introns and exons and the potential to produce many different mature RNA molecules from a single gene.
★ WJEC A Level Biology Specification Reference: - DNA contains the code for proteins and that between the exons are regions of non-coding DNA called introns.
★ AQA A Level Biology Specification Reference: - 3.4.2 DNA and protein synthesis - The structure and function of messenger RNA (mRNA) and of transfer RNA (tRNA).
★ CIE A Level Biology Specification Reference: - 6 Nucleic acids and protein synthesis. Explain the role of messenger RNA (mRNA), transfer RNA (tRNA) and the ribosomes.
★ Edexcel A Level Biology (Biology A – Salters-Nuffield) Specification Reference: - Topic 2: Genes and Health: 2.6 i) Understand the process of protein synthesis (transcription) including the role of RNA polymerase, translation, messenger RNA, transfer RNA, ribosomes and the role of start and stop codons. ii) Understand the roles of the DNA template (antisense) strand in transcription, codons on messenger RNA and anticodons on transfer RNA.
★ Edexcel A Level Biology (Biology B) Specification Reference: - Know the structure of mRNA including nucleotides, the sugar phosphate backbone and the role of hydrogen bonds. Know the structure of tRNA, including nucleotides, the role of hydrogen bonds and the anticodon.
★ OCR A Level Biology (Biology A) Specification Reference: - 2.1.3 Nucleotides and nucleic acids: transcription and translation of genes resulting in the synthesis of polypeptides. To include, the roles of RNA polymerase, messenger (m)RNA, transfer (t)RNA, ribosomal (r)RNA.
★ OCR A Level Biology (Biology B) Specification Reference: -2.1.4 Nucleic acids - To include, the roles of messenger (m)RNA, transfer (t)RNA, and ribosomal (r)RNA.
★ WJEC A Level Biology Specification Reference: - Core Concepts 5. Nucleic acids and their functions: -(f) the similarities and differences in the structure of RNA and DNA. (l) the transcription of DNA to produce messenger RNA. (m) the translation of mRNA using ribosomes and the structure and function of transfer RNA, to synthesise proteins. (Structure and Function of mRNA and tRNA).
★ AQA A Level Biology Specification Reference: - 3.1.5 Nucleic acids are important information-carrying molecules. 3.1.5.1 Structure of DNA. 3.4.1 DNA, genes and chromosomes: In prokaryotic cells, DNA molecules are short, circular and not associated with proteins. In the nucleus of eukaryotic cells, DNA molecules are very long, linear and associated with proteins, called histones. Together a DNA molecule and its associated proteins form a chromosome. The mitochondria and chloroplasts of eukaryotic cells also contain DNA which, like the DNA of prokaryotes, is short, circular and not associated with protein. A gene is a base sequence of DNA that codes for: the amino acid sequence of a polypeptide a functional RNA (including ribosomal RNA and tRNAs). A gene occupies a fixed position, called a locus, on a particular DNA molecule. A sequence of three DNA bases, called a triplet, codes for a specific amino acid. The genetic code is universal, non-overlapping and degenerate. In eukaryotes, much of the nuclear DNA does not code for polypeptides. There are, for example, non-coding multiple repeats of base sequences between genes. Even within a gene only some sequences, called exons, code for amino acid sequences. Within the gene, these exons are separated by one or more non-coding sequences, called introns.
★ CIE A Level Biology Specification Reference: - 6 Nucleic acids. 6.1 Structure of DNA. 1.2 Cells as the basic units of living organisms: d) outline key structural features of typical prokaryotic cells as seen in a typical bacterium (including: unicellular, 1–5μm diameter, peptidoglycan cell walls, lack of membrane-bound organelles, naked circular DNA, 70S ribosomes). a) describe the structure of a chromosome, limited to DNA, histone proteins, chromatids, centromere and telomeres. mitochondria (including small circular DNA). Chloroplasts (including small circular DNA).
★ Edexcel A Level Biology (Biology A – Salters-Nuffield) Specification Reference: - Topic 2: Genes and Health: 2.8 Know that a gene is a sequence of bases on a DNA molecule that codes for a sequence of amino acids in a polypeptide chain. Topic 3: Voice of the Genome: 3.4 Know the ultrastructure of prokaryotic cells, including cell wall, capsule, plasmid, flagellum, pili, ribosomes, mesosomes and circular DNA.
★ Edexcel A Level Biology (Biology B) Specification Reference: - Know that a gene is a sequence of bases on a DNA molecule coding for a sequence of amino acids in a polypeptide chain. v Know the ultrastructure of eukaryotic cells and the functions of organelles, including: nucleus, nucleolus, mitochondria.
★ OCR A Level Biology (Biology A) Specification Reference: - The Structure of DNA. The structure of a chloroplast: The components of a chloroplast including outer membrane, lamellae, grana, thylakoid, stroma and DNA. The structure of the mitochondrion - The components of a mitochondrion including inner and outer mitochondrial membranes, cristae, matrix and mitochondrial DNA.
★ OCR A Level Biology (Biology B) Specification Reference: - 2.1.1 Cells and microscopy (i) the ultrastructure of a typical eukaryotic plant cell such as a palisade mesophyll cell and a prokaryotic cell, as revealed by an electron microscope. To include the structure and function of the mitochondria, nucleus and nucleolus. The ultrastructure a prokaryotic cell, as revealed by an electron microscope. The structure and function of chloroplasts, circular DNA.
★ WJEC A Level Biology Specification Reference: - DNA contains the code for proteins and that between the exons are regions of non-coding DNA called introns. The structure and function of the mitochondria; chloroplasts.
★ AQA A Level Biology Specification Reference: - 3.8.4.1 Recombinant DNA technology (A-level only). Recombinant DNA technology involves the transfer of fragments of DNA from one organism, or species, to another. Since the genetic code is universal, as are transcription and translation mechanisms, the transferred DNA can be translated within cells of the recipient (transgenic) organism. Interpret information relating to the use of recombinant DNA technology.
★ CIE A Level Biology Specification Reference: - 19.1 Principles of genetic Technology: a) define the term recombinant DNA. b) explain that genetic engineering involves the extraction of genes from one organism, or the synthesis of genes, in order to place them in another organism (of the same or another species) such that the receiving organism expresses the gene product.
★ Edexcel A Level Biology (Biology A – Salters-Nuffield) Specification Reference: -
★ Edexcel A Level Biology (Biology B) Specification Reference: - Topic 7: Modern Genetics. 7.4 Gene technology: Understand how recombinant DNA can be produced.
★ OCR A Level Biology (Biology A) Specification Reference: - 6.1.3 Manipulating genomes (the principles of genetic engineering - Recombinant DNA).
★ OCR A Level Biology (Biology B) Specification Reference: - 5.1.3 Gene technologies.
★ WJEC A Level Biology Specification Reference: - 7. Application of reproduction and genetics.
★ AQA A Level Biology Specification Reference: - 3.8.4.1 Recombinant DNA technology (A-level only). Recombinant DNA technology involves the transfer of fragments of DNA from one organism, or species, to another. Since the genetic code is universal, as are transcription and translation mechanisms, the transferred DNA can be translated within cells of the recipient (transgenic) organism. Fragments of DNA can be produced by: Conversion of mRNA to complementary DNA (cDNA), using reverse transcriptase.
★ CIE A Level Biology Specification Reference: - 19.1 Principles of genetic Technology: h) explain the role of reverse transcriptase in genetic engineering.
★ OCR A Level Biology (Biology A) Specification Reference: - 6.1.3 Manipulating genomes (f) the principles the principles of genetic engineering. To include the isolation of genes from one organism and the placing of these genes into another organism using suitable vectors. NOTE: cDNA and using reverse transcriptase is not specifically mentioned in the specification!
★ OCR A Level Biology (Biology B) Specification Reference: - 5.1.3 Gene technologies (b) To include the role of reverse transcriptase - example of human protein to include insulin.
★ Edexcel A Level Biology (Biology A – Salters-Nuffield) N/A
★ Edexcel A Level Biology (Biology B) N/A
★ WJEC A Level Biology N/A
★ AQA A Level Biology Specification Reference: - 3.8.4 Gene technologies. Fragments of DNA can be amplified by in vitro and in vivo techniques - The principles of the polymerase chain reaction (PCR) as an in vitro method to amplify DNA fragments.
★ CIE A Level Biology Specification Reference: - 19.1 Principles of genetic technology. c) describe the principles of the polymerase chain reaction (PCR) to clone and amplify DNA (the role of Taq polymerase (the DNA Polymerase enzyme from the heat tolerant bacterium Thermus aquaticus) should be emphasised).
★ Edexcel A Level Biology (Biology A – Salters-Nuffield) Specification Reference: - Topic 6: Immunity, Infection and Forensics. 6.4 Know how DNA can be amplified using the polymerase chain reaction (PCR).
★ Edexcel A Level Biology (Biology B) Specification Reference: - Topic 7: Modern Genetics. 7.1 Using gene sequencing. ii Understand how PCR can be used to amplify DNA samples, and how these samples can be used: e.g. to predict the amino acid sequence of proteins and possible links to genetically determined conditions, using gene sequencing / in forensic science, to identify criminals and to test paternity, using DNA profiling.
★ OCR A Level Biology (Biology A) Specification Reference: - 6.1.3 Manipulating genomes. (d) the principles of the polymerase chain reaction (PCR) and its application in DNA analysis.
★ OCR A Level Biology (Biology B) Specification Reference: - 5.1.3 Gene technologies. The principles and uses of the Polymerase Chain Reaction (PCR). To include the use of PCR in amplifying DNA, the role of primers and Taq polymerase ((the DNA Polymerase enzyme from the heat tolerant bacterium Thermus aquaticus) in PCR.
★ WJEC A Level Biology Specification Reference: - 7. Application of reproduction and genetics. (d) the use of PCR and electrophoresis to produce a genetic fingerprint; the forensic use of genetic fingerprinting.
★ AQA A Level Biology Specification Reference: - 3.8.4.3 Genetic fingerprinting (A-level only). An organism’s genome contains many variable number tandem repeats (VNTRs). The probability of two individuals having the same VNTRs is very low. The technique of genetic fingerprinting in analysing DNA fragments that have been cloned by PCR, and its use in determining genetic relationships and in determining the genetic variability within a population. The use of genetic fingerprinting in the fields of forensic science, medical diagnosis, animal and plant breeding. Students should be able to: Explain the biological principles that underpin genetic fingerprinting techniques. Interpret data showing the results of gel electrophoresis to separate DNA fragments. Explain why scientists might use genetic fingerprinting in the fields of forensic science, medical diagnosis, animal and plant breeding.
★ CIE A Level Biology Specification Reference: - 19.1 Principles of genetic technology. Describe and explain how gel electrophoresis is used to analyse proteins and nucleic acids, and to distinguish between the alleles of a gene (limited to the separation of polypeptides and the separation of DNA fragments cut with restriction endonucleases). 19.2 Genetic technology applied to medicine outline the use of PCR and DNA testing in forensic medicine and criminal investigations.
★ Edexcel A Level Biology (Biology A – Salters-Nuffield) Specification Reference: - Topic 6: Immunity, Infection and Forensics. 6.3 Know how DNA profiling is used for identification and determining genetic relationships between organisms (plants and animals).
★ Edexcel A Level Biology (Biology B) Specification Reference: - 7.1 Using gene sequencing. ii Understand how PCR can be used to amplify DNA samples, and how these samples can be used: in forensic science, to identify criminals and to test paternity, using DNA profiling.
★ OCR A Level Biology (Biology A) Specification Reference: - 6.1.3 Manipulating genomes. The principles of DNA sequencing and the development of new DNA sequencing techniques. The principles of DNA profiling and its uses, to include forensics and analysis of disease risk. the principles of the polymerase chain reaction (PCR) and its application in DNA analysis. the principles and uses of electrophoresis for separating nucleic acid fragments or proteins.
★ OCR A Level Biology (Biology B) Specification Reference: - 5.1.3 Gene technologies. The principles and uses of the Polymerase Chain Reaction (PCR). The nature and use VNTRs (variable number tandem repeats) in human genome studies. To include forensics, disease pre-disposition, ethnic migration, paternity testing, selection for clinical trials.
★ WJEC A Level Biology Specification Reference: - 7. Application of reproduction and genetics. This topic covers gene technology and its applications, including the sequencing of genomes, the use of PCR and recombinant DNA technology. Learners should be able to demonstrate and apply their knowledge and understanding of: the use of PCR and electrophoresis to produce a genetic fingerprint; the forensic use of genetic fingerprinting.
★ AQA A Level Biology Specification Reference: - 3.8.4.3 Genetic fingerprinting (A-level only). Students should be able to: interpret data showing the results of gel electrophoresis to separate DNA fragments.
★ CIE A Level Biology Specification Reference: - 19.1 Principles of genetic Technology: d) describe and explain how gel electrophoresis is used to analyse proteins and nucleic acids, and to distinguish between the alleles of a gene (limited to the separation of polypeptides and the separation of DNA fragments cut with restriction endonucleases)
★ Edexcel A Level Biology (Biology A – Salters-Nuffield) Specification Reference: - Topic 6: Immunity, Infection and Forensics. Use gel electrophoresis to separate DNA fragments of different length.
★ Edexcel A Level Biology (Biology B) Specification Reference: - N/A. Note; Electrophoresis is NOT specifically mentioned in the spec. but understanding it is essential for Topic 7: Modern Genetics. 7.1 Using gene sequencing - in particular in forensic science, to identify criminals and to test paternity, using DNA profiling.
★ OCR A Level Biology (Biology A) Specification Reference: - 6.1.3 Manipulating genomes (e) the principles and uses of electrophoresis for separating nucleic acid fragments or proteins
★ OCR A Level Biology (Biology B) Specification Reference: - 5.1.3 Gene technologies (d) the principles and uses of agarose gel electrophoresis.
★ WJEC A Level Biology Specification Reference: - 7. Application of reproduction and genetics: (d) the use of PCR and electrophoresis to produce a genetic fingerprint; the forensic use of genetic fingerprinting.
★ AQA A Level Biology Specification Reference: - 3.7.1 Inheritance (A-level only). In a diploid organism, the alleles at a specific locus may be either homozygous or heterozygous. Monohybrid and cross involving dominant, recessive and alleles. Students could use information to represent phenotypic ratios in monohybrid crosses. Students could show understanding of the probability associated with inheritance. 3.7.2 Populations (A-level only). The Hardy–Weinberg principle provides a mathematical model, which predicts that allele frequencies will not change from generation to generation. The conditions under which the principle applies. The frequency of alleles, genotypes and phenotypes in a population can be calculated using the Hardy–Weinberg equation. Students could collect data about the frequency of observable phenotypes within a single population. Students could calculate allele, genotype and phenotype frequencies from appropriate data using the Hardy–Weinberg equation.
★ CIE A Level Biology Specification Reference: - 17.2 Natural and artificial: d) Use the Hardy–Weinberg principle to calculate allele, genotype and phenotype frequencies in populations and explain situations when this principle does not apply selection.
★ Edexcel A Level Biology (Biology A – Salters-Nuffield) Specification Reference: - Topic 2: Genes and Health. 2.13 i) Know the meaning of the terms: gene, allele, genotype, phenotype, recessive, dominant, homozygote and heterozygote. ii) Understand patterns of inheritance, including the interpretation of monohybrid inheritance. Topic 4: Biodiversity and Natural Resources: 4.5 i) Understand how the Hardy-Weinberg equation can be used to see whether a change in allele frequency is occurring in a population over time.
★ Edexcel A Level Biology (Biology B) Specification Reference: - 8.2 Transfer of genetic information: Understand the terms ‘genotype and phenotype’,’ homozygote and heterozygote’, ‘dominance’, ‘recessive’. Be able to construct genetic crosses. 8.3 Gene pools - Understand how the Hardy-Weinberg equation can be used to monitor changes in the allele frequencies in a population.
★ OCR A Level Biology (Biology A) Specification Reference: - 6.1.2 Patterns of inheritance. (f) the use of the Hardy–Weinberg principle to calculate allele frequencies in populations. The equations for the Hardy–Weinberg principle will be provided where needed in assessments and do not need to be recalled.
★ OCR A Level Biology (Biology B) Specification Reference: - 5.1.2 Population genetics and epigenetics. (c) the use of Hardy-Weinberg equations to analyse changes in allele frequencies in populations. The equations for the Hardy-Weinberg principle will be provided where needed in assessments and do not need to be recalled.
★ WJEC A Level Biology Specification Reference: - Continuity of Life: 6. Variation and evolution. (g) the use of the Hardy-Weinberg principle and equation. (h) the conditions under which the Hardy-Weinberg principle applies.