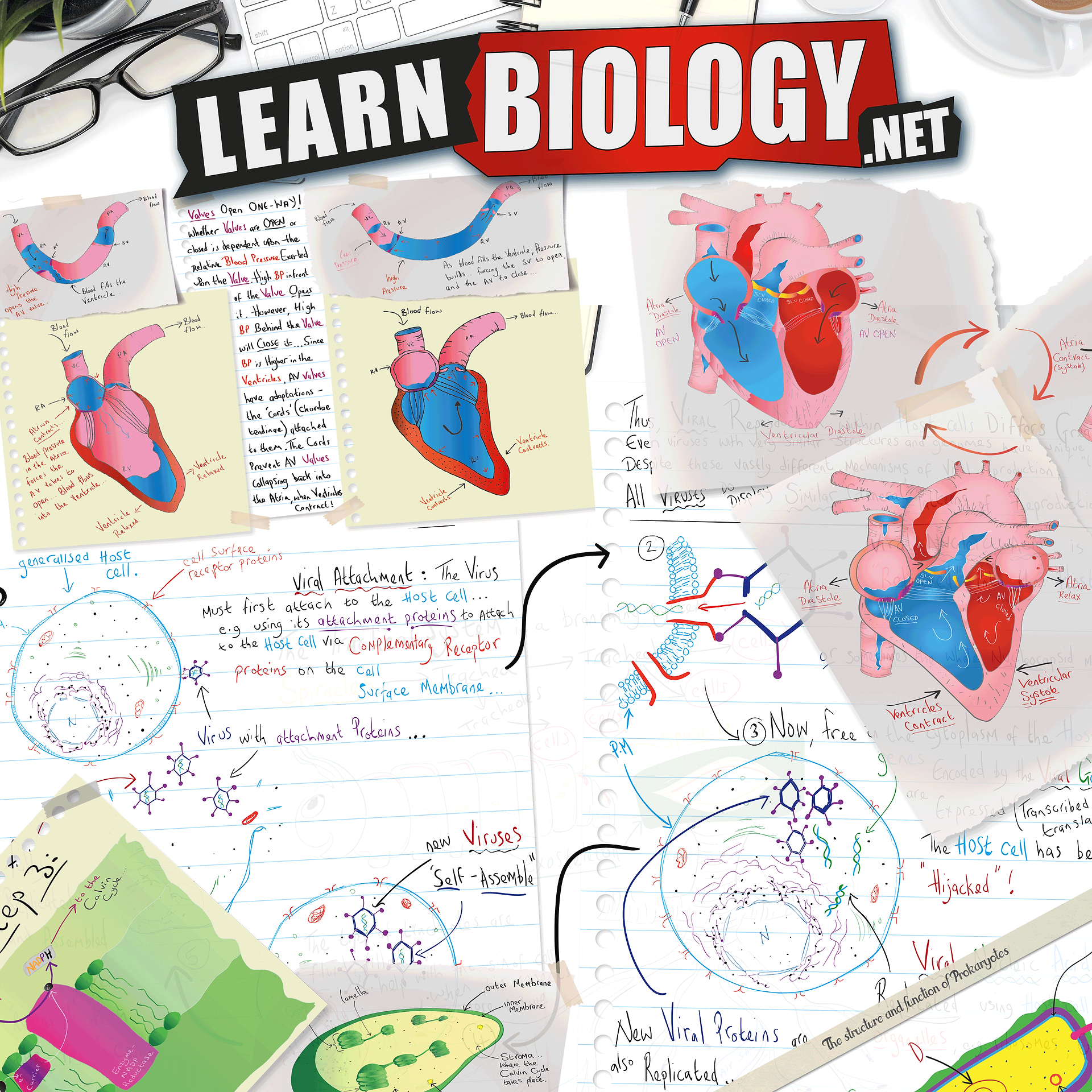
Become a site member today and access 100's of fantastic biology resources, including interactive workbooks with detailed answers, quick revision sheets to reinforce key concepts and revision videos to help you learn key concepts quickly and effectively.
The Cardiovascular system (summary)
The Heart
The Heart Valves
Arteries
Capillaries
Veins
The Cardiac cycle
The Cardiac cycle (graph interpretation)
The formation of Tissue Fluid
The Respiratory System
The Digestive System
The Nervous System
The Cardiovascular System
In both GCSE and A-level biology you’ll be expected to compare and contrast mass transport systems. Here, we’ll take a look at the the mammalian cardiovascular system (circulatory system).
When organisms develops organs and organ systems in order to maximise exchange (i.e. gas exchange systems), these organisms also need a mass transport system – a network of vessels circulating and connecting large volumes of fluid (ie. blood) and dissolved substances to organs and tissues around the body.
This transport system is also known as a mass flow system. For example, many plants have vascular systems composed of the xylem and phloem; Mammals (like Humans) and other animals have circulatory systems composed of the heart and blood vessels (arteries, capillaries and veins).
Mammals are endothermic animals with a high metabolic rate. Which means an efficient circulatory system is needed in order to deliver large amounts of raw materials (e.g. oxygen and glucose) to cells, tissues and organs. The system must also take away waste products (e.g. carbon dioxide). In order to do this efficiently, mammals have a double circulatory system i.e. a pulmonary system and a systemic circulatory system.
What is pulmonary circulation?
Pulmonary: - pulmonary = lungs.
Circulation: - movement of fluids in a closed system.
Pulmonary circulation takes blood on a relatively short return journey to and from the the lungs.
When gas exchange takes place in the alveoli, pressure is lost. So, oxygenated blood must now be transported to the heart (our muscular pump) which pumps oxygenated blood around the body (systemic circulation).
What is Systemic circulation?
Systemic: - the ‘body’ / organism.
Circulation: -movement of fluids in a closed system.
In systemic circulation the heart pumps blood to the body. Blood is transported through a series of ever decreasing blood vessels (arteries - arterioles - capillaries). When blood passes through a system of capillary beds at tissues and exchange takes place pressure is lost once more. Following the exchange of materials (e.g. oxygen supplied to tissues and the waste product carbon dioxide picked up by the blood). Now, the deoxygenated blood must return to the heart, where the necessary ‘pressure boost’ pumps the deoxygenated blood to the lungs and the cycle continues.
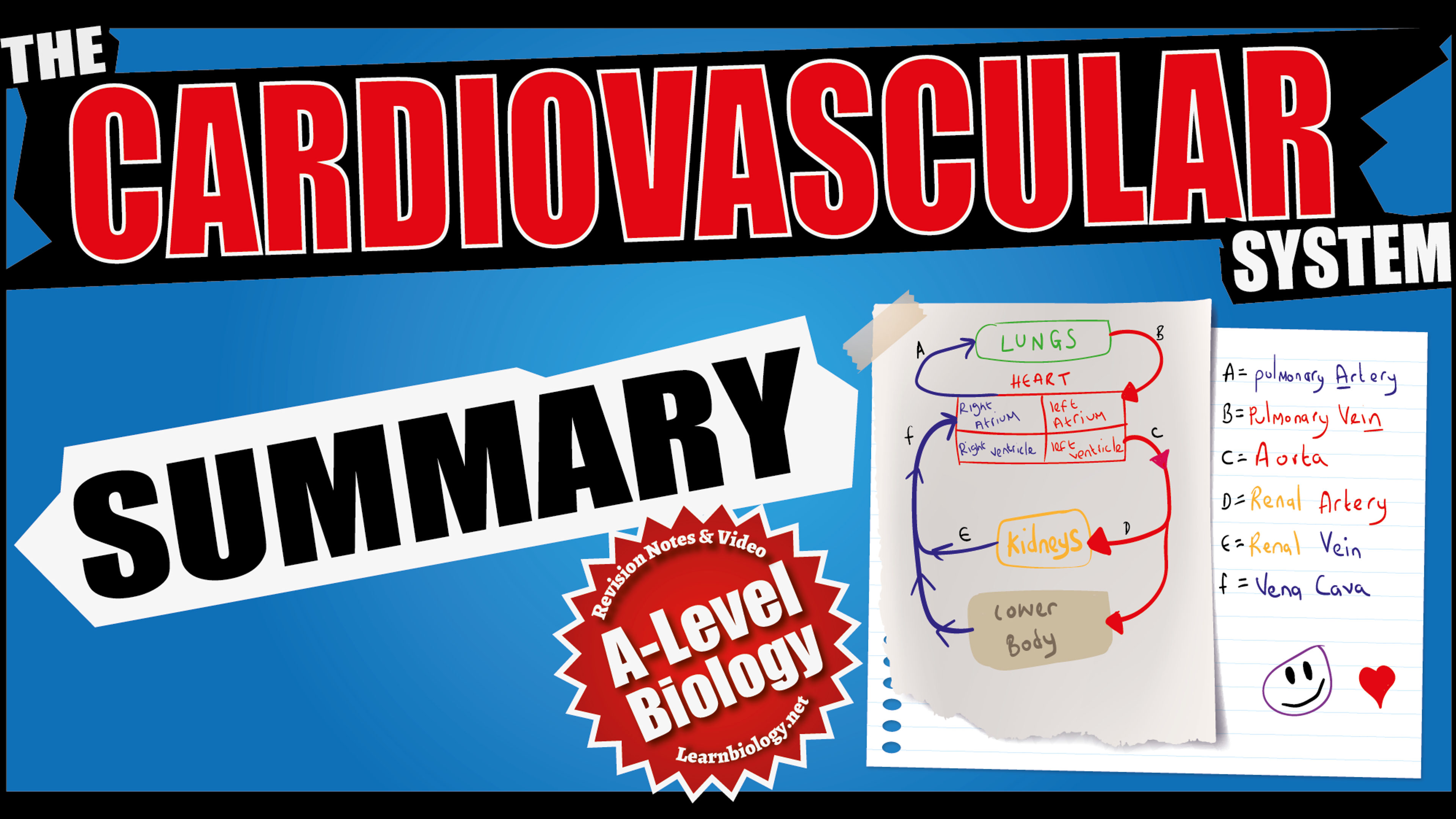
Human Circulatory System:
Small organisms don’t have a bloodstream, but instead rely on the simple diffusion of materials for transport around their cells. This is OK for single cells, but it would take days for molecules to diffuse through a large animal, so most animals have a circulatory system with a pump to transport materials quickly around their bodies. This is an example of a mass flow system (or mass transport system), which means the transport of substances in the flow of a fluid (as opposed to diffusion, which is the random motion of molecules in a stationary fluid).
The transport of materials in the xylem and phloem of plants is an other example of mass flow.
Mass flow systems work together with the specialised exchange systems (such as lungs, gills and leaves). Humans, however have a double circulatory system with a 4-chambered heart. In humans the right side of the heart pumps blood to the lungs only and is called the pulmonary circulation, while the left side of the heart pumps blood to the rest of the body – the systemic circulation.
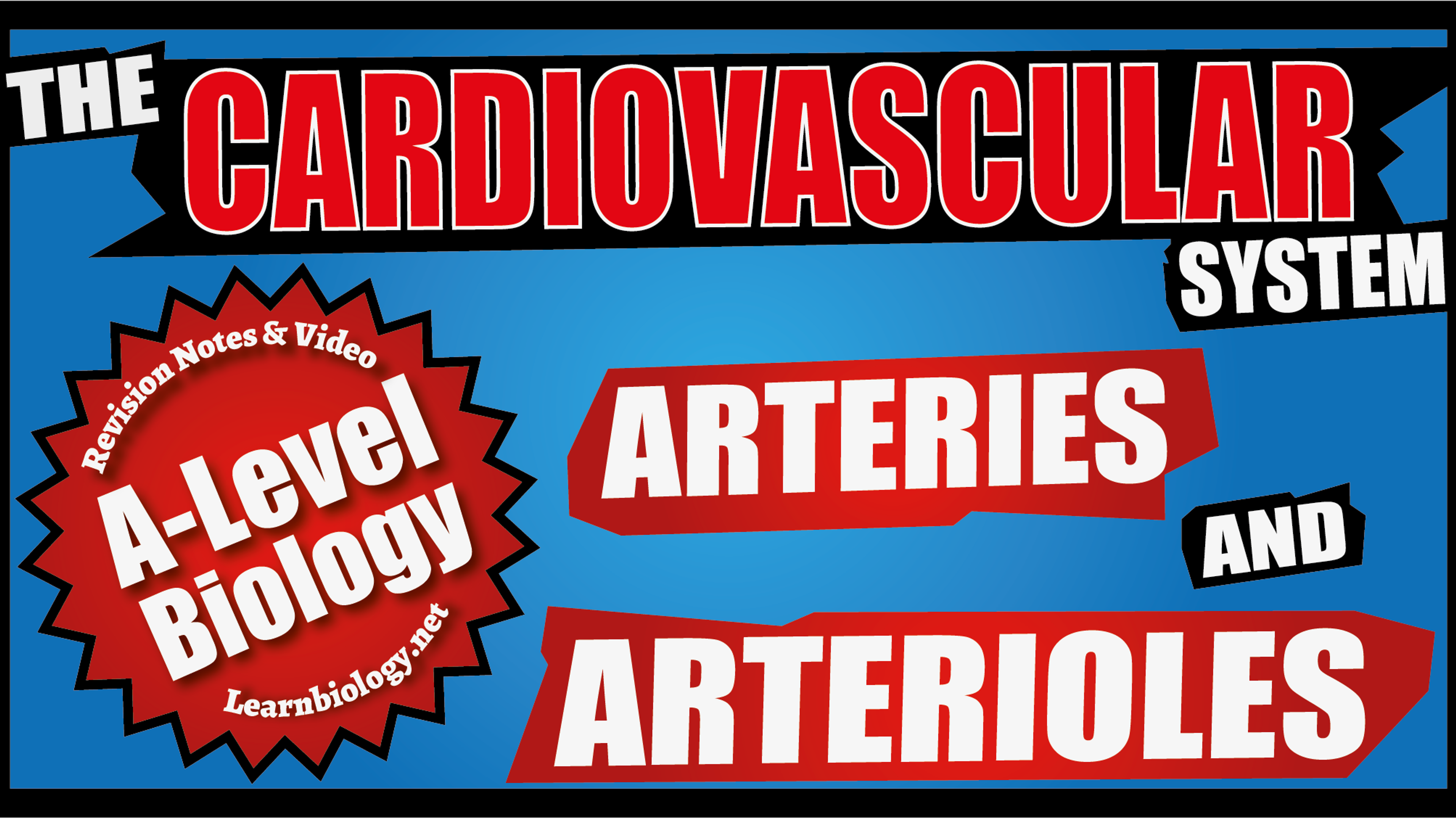
Blood vessels
Blood circulates through a series of different kinds of blood vessels as it circulates around the body.
Each kind of vessel is adapted to its function.
Arteries carry blood from the heart to every tissue in the body. Arteries have thick, elastic walls to withstand the high pressure of blood from the heart.
The arteries close to the heart are particularly elastic and expand during systole and recoil again during diastole, helping to even out the pulsating blood flow. The smaller arteries and arterioles are more muscular and can contract (vasoconstriction) to close off the capillary beds to which they lead; or relax (vasodilation) to open up the capillary bed. These changes are happening constantly under the involuntary control of the medulla in the brain, and are most obvious in the capillary beds of the skin, causing the skin to change colour from pink (skin arterioles dilated) to blue (skin arterioles constricted). There is not enough blood to fill all the body’s capillaries, and at any given time up to 20% of the capillary beds are closed off.
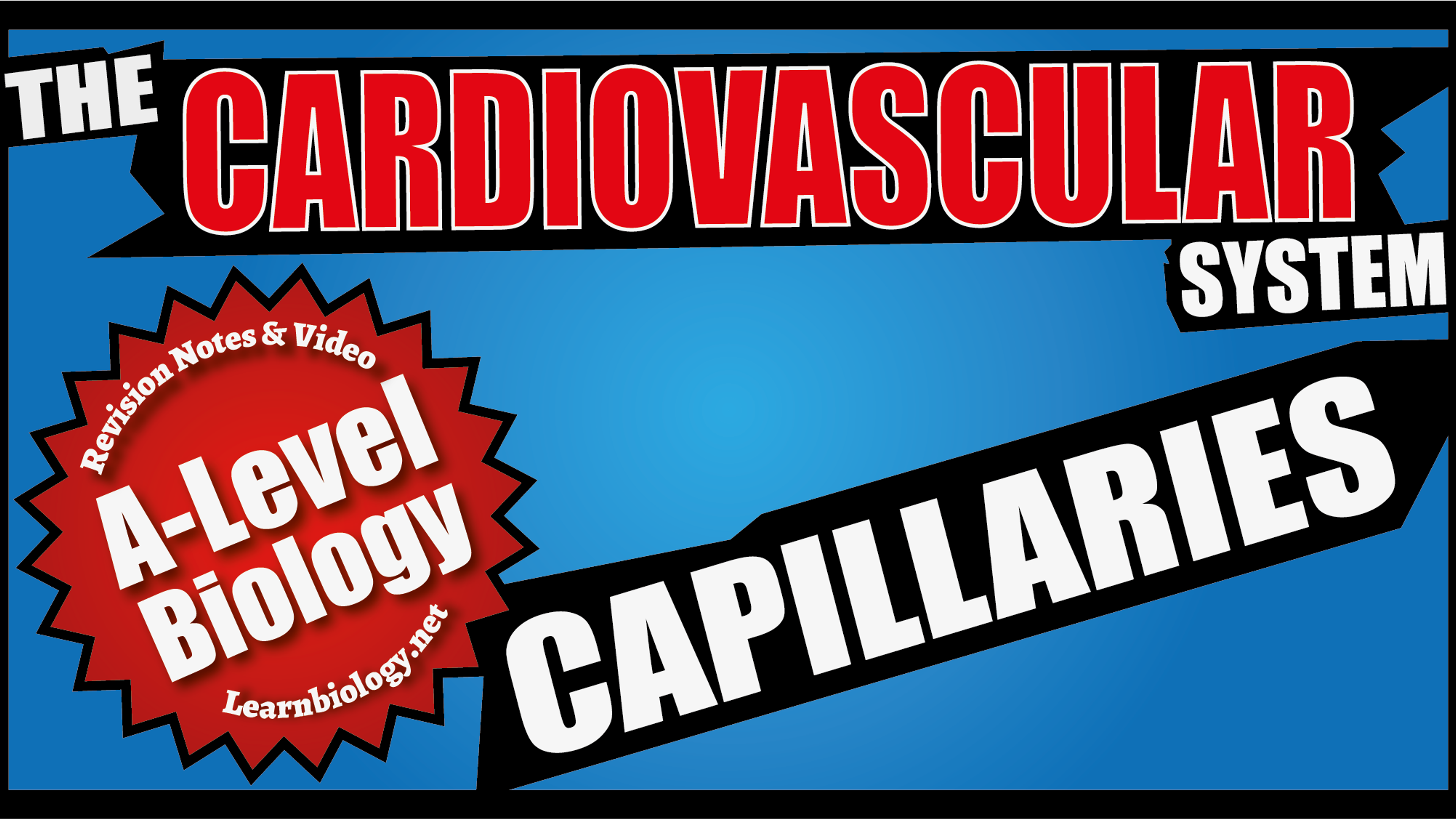
Capillaries are where the transported substances actually enter and leave the blood. No exchange of materials takes place in the arteries and veins, whose walls are too thick and impermeable.
Capillaries are very narrow and thin-walled, but there are a vast number of them (108 m in one adult!). Capillaries have a Large surface area : Volume ratio, helping rapid diffusion of substances between blood and cells. Capillaries are arranged in networks called capillary beds, which feed a group of cells, and no cell in the body is more than 2 cells away from a capillary.
Veins carry blood from every tissue in the body to the heart. The blood has lost almost all its pressure in the capillaries, so it is at low pressure inside veins and moving slowly.
Veins therefore don’t need thick walls and they have a larger lumen that arteries, to reduce the resistance to flow.
Veins also have semi-lunar valves to stop the blood flowing backwards. It is particularly difficult for blood to flow upwards through the legs to heart, and the flow is helped by contractions of the leg and abdominal muscles: [insert diagram showing valves].
The body relies on constant contraction of these muscles to get the blood back to the heart, and this explains why soldiers standing still on parade for long periods can faint, and why sitting still on a long flight can cause swelling of the ankles and Deep Vein Thrombosis (DVT or “economy class syndrome”), where small blood clots collect in the legs.
The Human Heart:
The human heart has four chambers: two thin-walled atria on top, which receive blood, and two thick walled ventricles beneath the atria. the thick walled ventricles ‘pump’ blood.
Veins carry blood into the atria and arteries carry blood away from the ventricles.
Between the atria and the ventricles are atrioventricular valves, which prevent back-flow of blood from the ventricles to the atria.
The left valve has two flaps and is called the bicuspid (or mitral) valve, while the right valve has 3 flaps and is called the tricuspid valve. The valves are held in place by valve tendons attached to papillary muscles, which contract at the same time as the ventricles, holding the valves closed.
There are also two semi-lunar valves in the arteries (the only examples of valves in arteries) called the pulmonary and aortic valves.
The left and right halves of the heart are separated by the inter-ventricular septum. The walls of the right ventricle are 3 times thinner than on the left and it produces less force and pressure in the blood. This is in part due to the blood having less distance to travel (the lungs are right next to the heart), but also because a lower pressure in the pulmonary circulation means that less fluid passes from the capillaries to the alveoli.
The internal volume of the left and right ventricles is the same.
The heart is made of cardiac muscle, composed of cells called myocytes. When myocytes receive an electrical impulse they contract together, causing a heartbeat. Since myocytes are constantly active, they have a great requirement for oxygen, so are fed by numerous capillaries from two coronary arteries. Coronary arteries arise from the aorta as it leaves the heart. Deoxygenated Blood returns via the coronary sinus, which drains directly into the right atrium.
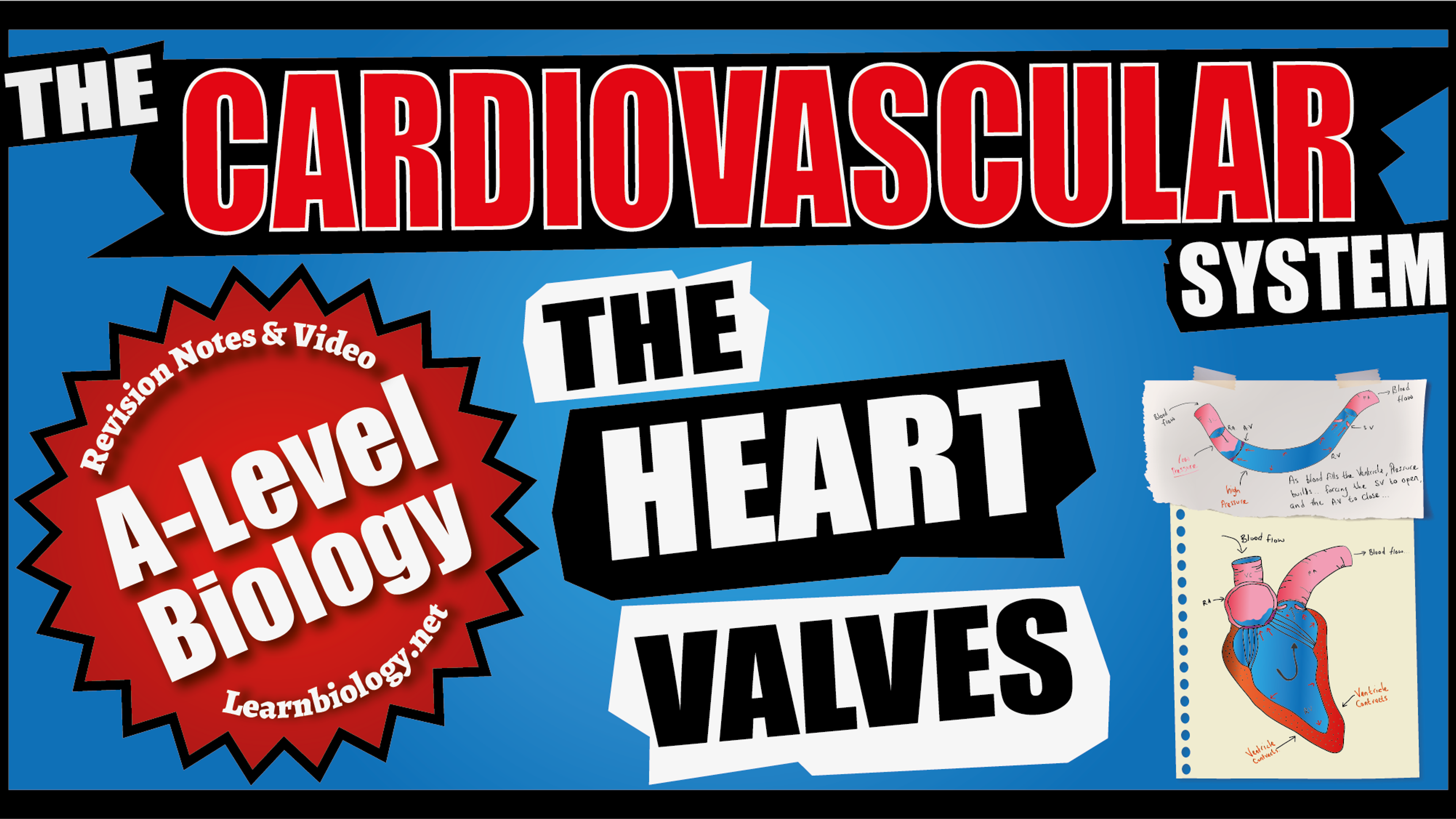
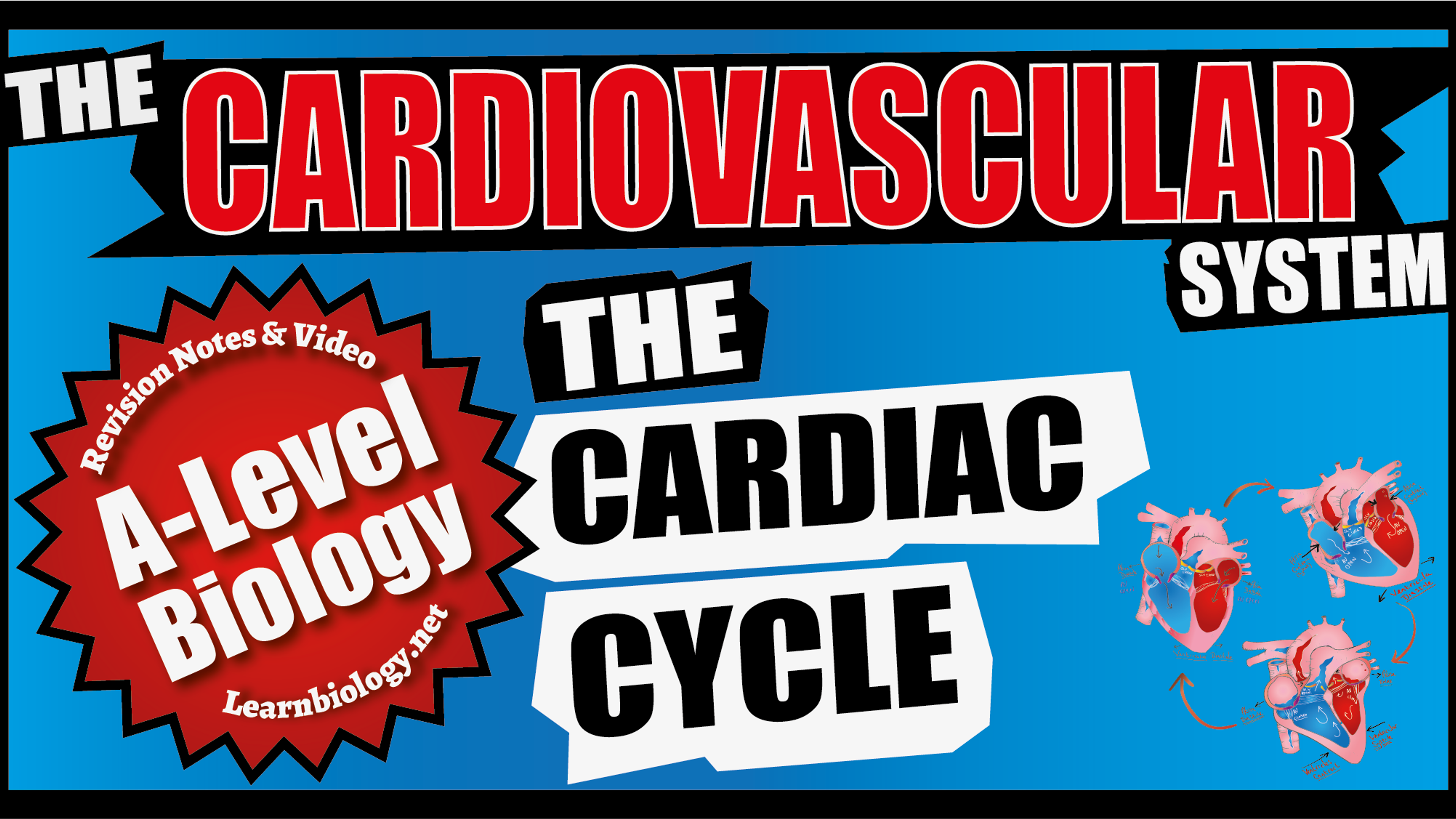
The Cardiac Cycle
Cardiac muscle contracts about 75 times per minute, pumping around 75 cm3 of blood from each ventricle each beat (the stroke volume).
Cardiac muscle is myogenic, which means that it can contract on its own, without needing nerve impulses. Contractions are initiated within the heart by the sino-atrial node (SAN, or pacemaker) in the right atrium. This extraordinary tissue acts as a clock, and contracts spontaneously and rhythmically about once a second, even when surgically removed from the heart.
[insert image of heart showing SAN, AVN Bundle of HIS and Purkinje fibres]
There is a complex sequence of events at each heartbeat called the cardiac cycle.
The cardiac cycle can be broken sown into three stages: -
1. Atrial Systole. The SAN contracts and transmits electrical impulses throughout the atria, which both contract, pumping blood into the ventricles. The ventricles are electrically insulated from the atria, so they do not contract at this time. The blood can't flow back into the veins because of the valves in the veins.
2. Ventricular Systole. The electrical impulse passes from the atrioventricular node (AVN) to the Purkinje (or Purkyne) fibres, with a short but important delay of about 0.1s. The Purkinje fibres pass down through the interventricular septum as the bundle of His, which is insulated from the surrounding muscle cells, so the ventricles do not contract yet. At the base of the ventricles the Purkinje fibres spread out and initiate ventricular contraction. The ventricles therefore contract shortly after the atria, from the bottom up, squeezing blood upwards into the arteries. The blood can't go into the atria because of the atrioventricular valves, which are forced shut with a "lub" sound.
3. Diastole. The atria and the ventricles relax, while the atria fill with blood. The semilunar valves in the arteries close as the arterial blood pushes against them, making a "dup" sound. The events of the three stages are shown in the chart below. The pressure changes show most clearly what is happening in each chamber. Blood flows because of pressure differences, and it always flows from a high pressure to a low pressure, if it can. So during atrial systole the atria contract, making the atrium pressure higher than the ventricle pressure, so blood flows from the atrium to the ventricle. The artery pressure is higher still, but blood can’t flow from the artery back into the heart due to the semi-lunar valves. The valves are largely passive: they are opened by blood flowing through them the right way and are forced closed when blood tries to flow through them the wrong way. Whenever lines cross in the pressure graph it means that a valve opens or closes.
The pressure changes show most clearly what is happening in each chamber. Blood flows because of pressure differences, and it always flows from a high pressure to a low pressure, if it can.
So, during atrial systole the atria contract, making the atrium pressure higher than the ventricle pressure, so blood flows from the atrium to the ventricle.
The artery pressure is higher still, but blood can’t flow from the artery back into the heart due to the semilunar valves. The valves are largely passive: they open when blood flows through them the right way and close when blood tries to flow through them the wrong way.
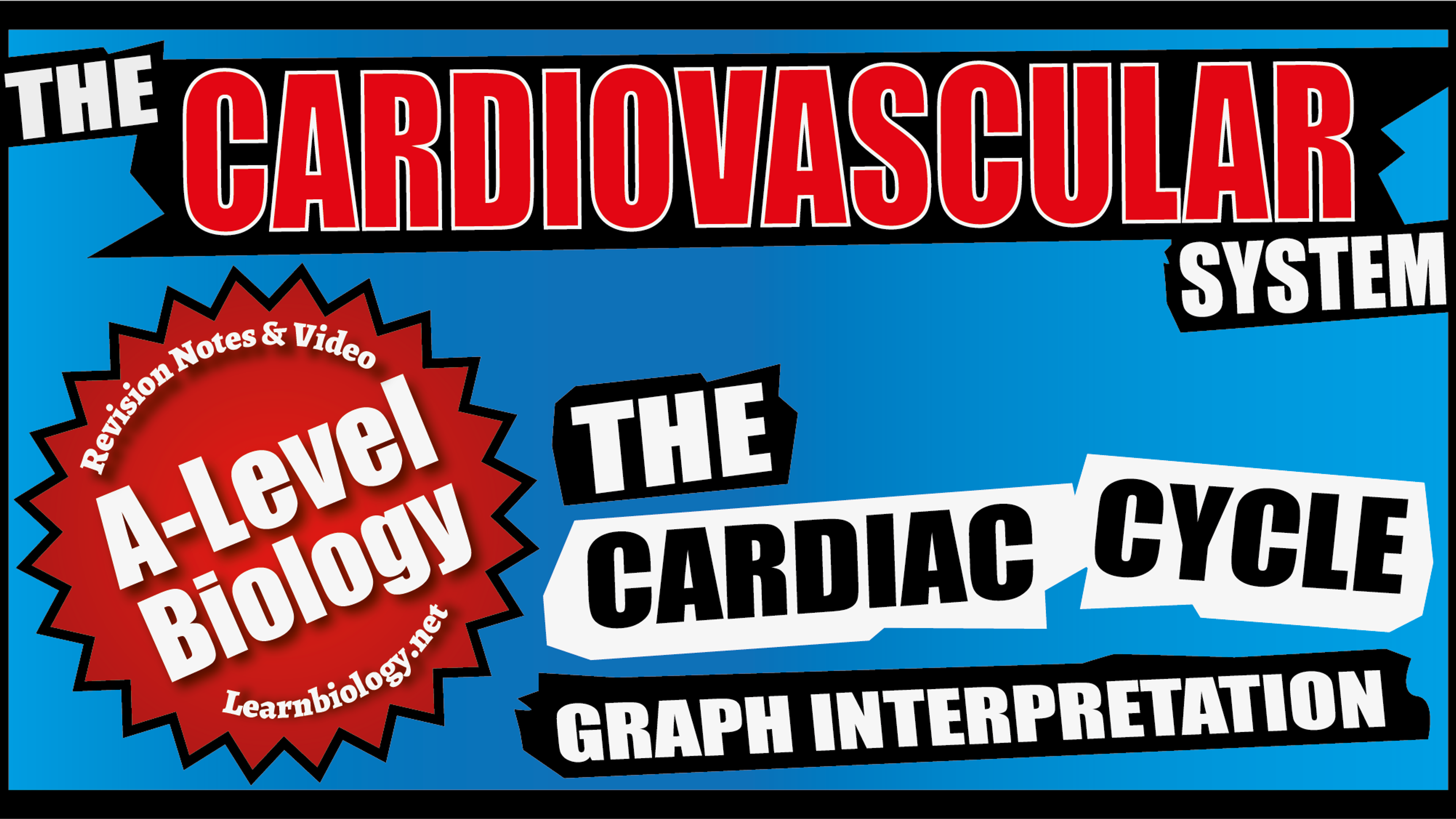
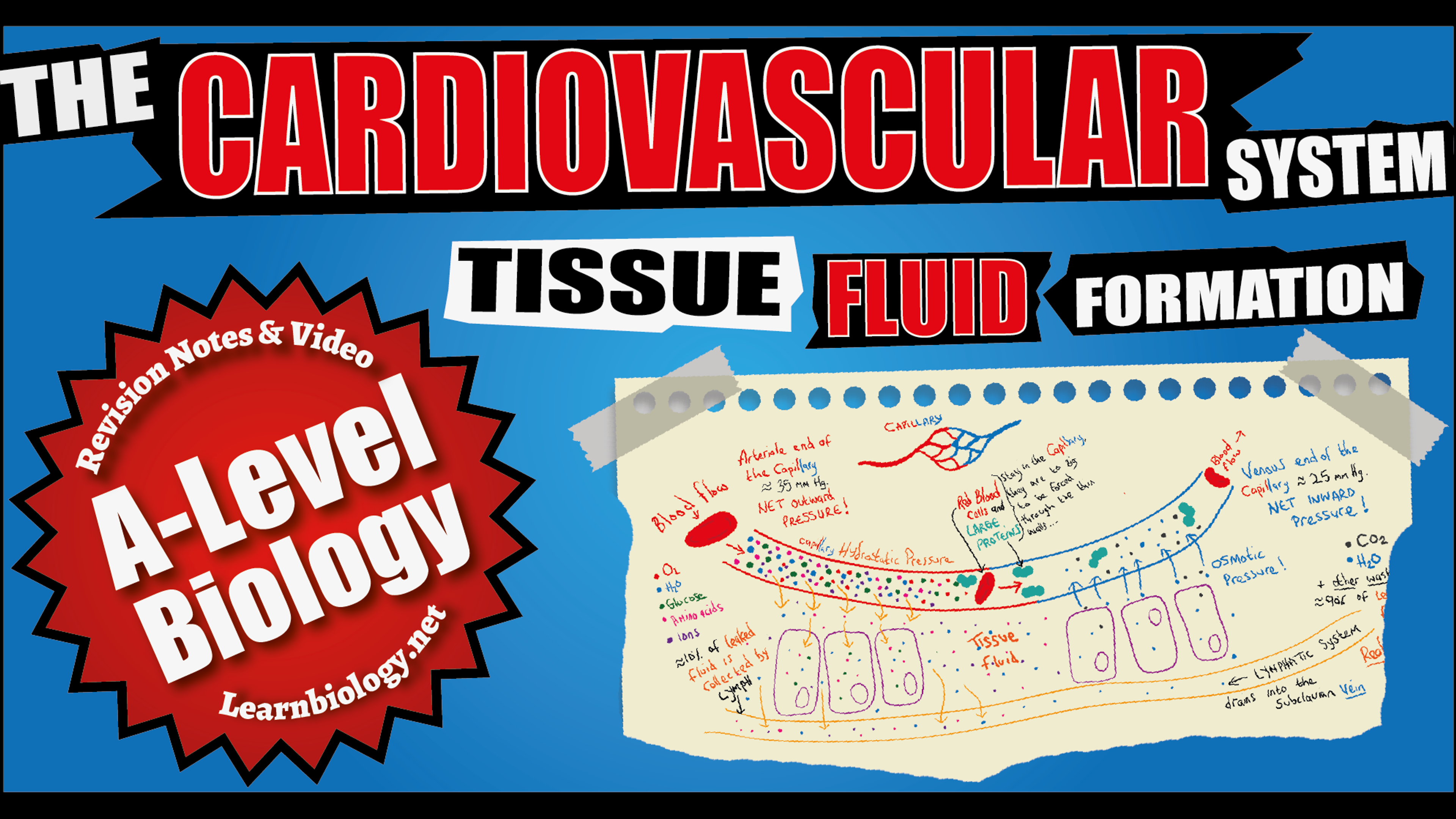
Tissue Fluid formation
The substances showing in the table above are all example of substances which are exchanged between the blood and the cells in capillary beds.
However! Substances do not actually move directly between the blood and the cell: they first diffuse into the tissue fluid that surrounds all cells, and then diffuse from tissue fluid to the cells!
So, how is tissue fluid formed?
1. At the arterial end of the capillary bed the blood is still at high hydrostatic pressure, so blood plasma is squeezed out through the permeable walls of the capillary. Cells and proteins are too big to leave the capillary, so they remain in the blood.
2. This fluid now forms tissue fluid surrounding the cells. Materials are exchanged between the tissue fluid and the cells by all four methods of transport across a cell membrane. Gases and lipid-soluble substances (such as steroids) cross by lipid diffusion; water crosses by osmosis, ions cross by facilitated diffusion; and glucose and amino acids cross by active transport.
3. At the venous end of the capillary bed the blood is at low pressure, since it has lost so much plasma. Water returns to the blood by osmosis since the blood has a low water potential. Solutes (such as carbon dioxide, urea, salts, etc) enter the blood by diffusion, down their concentration gradients.
4. Not all the plasma that left the blood returns to it, so there is excess tissue fluid. This excess drains into lymph vessels, which are found in all capillary beds. Lymph vessels have very thin walls, like capillaries, and tissue fluid can easily diffuse inside, forming lymph.
Blood
We have discussed quite a bit now about the heart and blood vessels transporting blood around the body. But what is Blood?
Blood is composed of 4 components, as shown in this diagram: [insert diagram of blood components]
1. Plasma - liquid part of blood. A dilute solution of salts, glucose, amino acids, vitamins, urea, proteins and fats.
2. White blood cells - involved in immune system.
3. Platelets - involved in blood clotting.
4. Red blood cells - involved in carrying oxygen.
There are many different substances in blood, all being transported from one part of the body to another. Some of the main ones are listed in this table
Transport of Oxygen
As you’ve learned above, one of the four components of blood are red blood cells, which carry oxygen around the body.
Oxygen is carried in red blood cells bound to a protein called haemoglobin.
Each red blood cell contains around 300 million haemoglobin molecules and there are around 5 million red blood cells per cm3 of blood!
The result is that blood can carry up to 20% oxygen (in comparison pure water can only carry 1%).
The haemoglobin molecule consists of four polypeptide chains, with a haem prosthetic group at the centre of each chain.
Each haem group contains one iron atom. One oxygen molecule binds to each iron atom.
So, one haemoglobin molecule can bind up to four oxygen molecules.
This means there are 4 binding steps as shown in this chemical equation:
[insert image showing chemical equation of oxygen binding]
A sample of blood can therefore be in any state from completely deoxygenated (0% saturated) to fully oxygenated (100% saturated).
Since deoxyhaemoglobin and oxyhaemoglobin are different colours, it is easy to measure the % saturation of a sample of blood in a colorimeter. As the chemical equation above shows, oxygen drives the reaction to the right, so the more oxygen there is in the surrounding environment, the more saturated the haemoglobin will be.
This relation is shown in an oxygen dissociation curve.
The concentration of oxygen in the surroundings can be measured as a % (there’s about 20% oxygen in air), but it’s more correct to measure it as a partial pressure (PO2, measured in kPa).
Luckily, since the pressure of one atmosphere is about 100 kPa, the actual values for PO2 and % O2 are the same (e.g. 12% O2 has a PO2 of 12 kPa).
The graph is read by starting with an oxygen concentration in the environment surrounding the blood capillaries on the X-axis, then reading off the state of the haemoglobin in the blood that results from the Y-axis.
This curve has an S (or sigmoid) shape, and shows several features that help in the transport of oxygen in the blood…
In the alveoli of the lungs oxygen is constantly being brought in by ventilation, so its concentration is kept high, at around 14 kPa. As blood passes through the capillaries surrounding the alveoli the haemoglobin binds oxygen to become almost 100% saturated. Even if the alveolar oxygen concentration falls a little the haemoglobin stays saturated because the curve is flat here.
In tissues, e.g. muscle, liver or brain, oxygen is used by respiration, so is low, typically about 4 kPa. At this PO2 the haemoglobin is only 50% saturated, so it unloads about half its oxygen (i.e. from about 100% saturated to about 50% saturated) to the cells, which use it for respiration.
In tissues that are respiring quickly, e.g. contracting muscle cells, the PO2 drops even lower, to about 2 kPa, so the haemoglobin saturation drops to about 10%, so almost 90% of the oxygen is unloaded, providing more oxygen for the muscle cells.
Actively-respiring tissues also produce a lot of CO2, which dissolves in tissue fluid to make carbonic acid which lowers the pH. The chemical equation above shows that hydrogen ions drive the reaction to the left, so low pH reduces the % saturation of haemoglobin at any PO2.
This is shown on the graph (above) by the dotted line, which is lower than the normal dissociation curve.
This downward shift is called the Bohr effect, (after the Danish scientist who first discovered it). So, at a PO2 of 2%, the actual saturation is nearer 5%, so almost all the oxygen loaded in the lungs is unloaded in respiring tissues.
It is important to remember that oxygen can only diffuse in and out of the blood from capillaries, which are permeable. Blood in arteries and veins is “sealed in”, so no oxygen can enter or leave the blood whatever the external conditions.
So as haemoglobin travels from the lungs to a capillary bed in a body tissue and back to the lungs, it “switches” from one position on the dissociation curve to another position, without experiencing the intermediate stages of the curve.
Transport of Carbon Dioxide
Carbon dioxide is carried between respiring tissues and the lungs by 3 different methods:
1. As dissolved gas in blood plasma (2%)
Very little travels this way as CO2 is not very soluble in water (about 0.02%)
2. As Carbamino Haemoglobin (13%)
Carbon dioxide can bind to amino groups in haemoglobin molecules, forming carbamate ions:
Since there are so many haemoglobin molecules in re blood cells, and each one has many amino groups, quite a lot of CO2 can be carried this way.
3. As Hydrogen Carbonate ions (85%)
Carbon dioxide reacts with water to form carbonic acid, which immediately dissociates to form a hydrogen carbonate (or bicarbonate) ion and a proton. This protons binds to haemoglobin, as in the cause of the Bohr effect. Hydrogen carbonate is very soluble, so most CO2 is carried this way. The reaction in water is very slow, but red blood cells contain the enzyme carbonic anhydrase, which catalyses the reaction with water by a factor of 108 times.
In respiring tissues CO2 produced by respiration diffuses into the red blood cells and forms hydrogen carbonate, which diffuses out of the cell into the blood plasma through an ion channel in the red blood cell membrane. This channel carries one chloride ion into the cell for every hydrogen carbonate ion it carries out, and this helps to keep the charge in the cell constant. In the lungs the reverse happens: hydrogen carbonate diffuses back into the red blood cell through the channel (and chloride goes out) and CO2 is formed by carbonic anhydrase (remember enzymes will catalyse reactions in either direction), which diffuses into the plasma and into the alveoli.
In all three cases of CO2 transport the direction of the reactions is governed by the concentration of CO2
So, in the tissues, where CO2 is high, the reactions go to the right, while in the lungs, where CO2 is low, the reactions go to the left.
Monitoring Heart activity:
A PCG (or phonocardiogram) is a recording of the sounds the heart makes. The cardiac muscle itself is silent and the sounds are made by the valves closing. The first sound (lub) is due to the atrioventricular valves closing and the second (dup) is due to the semi-lunar valves closing.
An ECG (or electrocardiogram) is a recording of the electrical activity of the heart. There are characteristic waves of electrical activity marking each phase of the cardiac cycle. Changes in these ECG waves can be used to help diagnose problems with the heart.
Cardiac Output:
Cardiac Output is the amount of blood flowing through the heart each minute.
You need to be able to calculate Cardiac Output (CO). which is calculated as the product of the Heart Rate and the Stroke Volume: -
CO = HR x SV
Heart Rate (HR) can be calculated from the pressure graph by measuring the time taken for one cardiac cycle. Use the formula to calculate HR: -
HR (beats per minute) = 60 / cycle time (seconds)
Stroke Volume:
The stroke volume is the volume of blood pumped in each beat.
Both heart rate and stroke volume can be varied by the body, for example when exercising the cardiac output can increase dramatically so that: -
-
oxygen and glucose can get to the muscles quicker
-
carbon dioxide and lactate can be carried away from the muscles quicker
-
heat can be carried away from the muscles quicker
Control of Heart Rate
You have learnt that the heart is myogenic – each heart beat is initiated by the sinoatrial node (SAN) in the heart itself (not by nerve impulses from the Central Nervous System (CNS). However, the heart rate and the stroke volume can both be controlled by the CNS to alter the cardiac output – the amount of blood flowing in a given time.
remember: Cardiac output = heart rate x stroke volume
Control of the heart rate is an involuntary reflex, and like many involuntary processes (such as breathing or sneezing) it is controlled by a region of the brain called the medulla. The medulla and its nerves are part of the autonomic nervous system (ANS).
The part of the medulla that controls the heart is called the cardiovascular centre (CVC). It receives inputs from various receptors around the body and sends output through two nerves to the sinoatrial node in the heart.
How does the cardiovascular centre control the heart?
The cardiovascular centre can control both the heart rate and the stroke volume. There are two separate nerves from the cardiovascular centre to the sinoatrial node: -
-
the sympathetic nerve to speed up the heart rate and
-
the parasympathetic nerve to slow it down.
The cardiovascular centre can also change the stroke volume by controlling blood pressure. It can increase the stroke volume by sending nerve impulses to the arterioles to cause vasoconstriction, which increases blood pressure so more blood fills the heart at diastole. Alternatively it can decrease the stroke volume by causing vasodilation and reducing the blood pressure.
How does the cardiovascular centre respond to exercise?
When the muscles are active they respire more quickly and cause several changes to the blood, such as decreased oxygen concentration, increased carbon dioxide concentration, decreased pH (since the carbon dioxide dissolves to form carbonic acid) and increased temperature. All of these changes are detected by various receptor cells around the body, but the pH changes are the most sensitive and therefore the most important. These pH changes are detected by chemoreceptors found in:-
-
The walls of the aorta (the aortic body), monitoring the blood as it leaves the heart
-
The walls of the carotid arteries (the carotid bodies), monitoring the blood to the head and brain
-
The medulla, monitoring the tissue fluid in the brain
The chemoreceptors send nerve impulses to the cardiovascular centre indicating that more respiration is taking place, and the cardiovascular centre responds by increasing the heart rate.
A similar job is performed by temperature receptors and stretch receptors in the muscles, which also detect increased muscle activity.
Coronary Heart Disease
Coronary heart disease (CHD) is caused by a blockage in the coronary blood system. This is the system that feeds the cardiac muscle cells so that they can respire and contract. Cardiac muscle works constantly throughout life and is incapable of anaerobic respiration, so it has a great demand for oxygen and glucose. There are two coronary arteries that arise directly from the aorta, and these split into numerous smaller arteries (arterioles) and then a network of capillaries, where exchange with the cardiac cells actually takes place. A blockage in a coronary artery can restrict the supply of oxygen to the cardiac cells, killing them and causing a heart attack. The steps are as follows…
1. Cholesterol and other insoluble lipids collect on the inside of a coronary artery. This deposit is called an atheroma, and it narrows the lumen of the artery, restricting blood flow – atherosclerosis.
2. The atheroma can collect minerals and become hardened to form a rough plaque.
3. The plaque weakens the wall of the artery, so the pressure of blood causes a local swelling called an aneurism. If the wall is particularly weak the aneurism may burst causing blood loss and probable death.
4. The plaque can also encourage the formation of a blood clot called a thrombus. Alternatively, a mobile clot from elsewhere in the blood stream (an embolism) can become lodged in the atheroma. The clot grows until it completely blocks the artery, forming a coronary thrombosis.
5. The thrombosis prevents oxygen reaching the cardiac cells “downstream” of the blockage, so they cannot respire and so die. This death of myocytes is a myocardial infarction, more commonly known as a heart attack. The severity of the heart attack depends on how far along the coronary artery the thrombosis is. If only a small part of one ventricle is killed then the patient will recover, but a thrombosis early in the coronary artery will always be fatal.
Myocardial Infarction (Heart Attack)
The five stages in a heart attack are summarised in this diagram.
-
Atheroma (fat deposits)
-
Plaque (hardened atheroma)
-
Aneurism (swelling due to weak wall)
-
Coronary Thrombosis (blockage due to blood clot)
-
Myocardial Infarction (death of cardiac cells)
Risk Factors for Coronary Heart Disease
There are a number of risk factors that are associated with coronary heart disease. The more of the factors that apply, the greater the risk of a heart attack. Some of the main factors are: -
Blood Cholesterol. Cholesterol in the blood comes from the diet and from the liver, where it is synthesised. Cholesterol is carried in large complexes with proteins, called lipoproteins. High-density lipoproteins (HDLs) remove cholesterol from tissues, so decrease the risk of atheromas, while low density lipoproteins (LDLs) deliver cholesterol to tissues, so increase the risk of atheromas.
Blood Pressure. High blood pressure increases the risk of an aneurism and stimulates thickening of artery wall, increasing the risk of thrombosis. Stress, diet and lack of exercise can all increase blood pressure.
Genetics. Both blood pressure and fat metabolism are affected by genes, so genes undoubtedly affect the chance of a coronary thrombosis. This doesn’t mean that, for some people, a heart attack is inevitable; it just means some people have to be even more careful about their lifestyle risk factors.
Diet. High levels of saturated fat increase the amount of cholesterol carried in the blood and so increase the risk of atherosclerosis. High levels of salt increase blood pressure and so increase the risk of aneurism. However, dietary fibre and vitamin C reduce the risk of heart disease.
Smoking. Smokers are between two and six times more likely to suffer from coronary heart disease than non-smokers. The carbon monoxide and nicotine in cigarette smoke both cause an increase in blood pressure.
Comparative Anatomy: Gas Exchange in Fish and Insects
A-Level Biology: Gas Exchange in Fish
Gas exchange is more difficult for fish than for mammals due to the concentration of dissolved
oxygen in water. Specifically dissolved O2 in H2O is less than 1%, compared to 20% in air. Incidentally, all animals need molecular oxygen for respiration and cannot break down water molecules to obtain oxygen.
Fish have developed specialised gas-exchange organs called gills, which are composed of thousands of filaments. The gill filaments are in turn are covered in feathery lamellae (each only a few cells thick). The Lamellae containing blood capillaries, where gas exchange takes place. Importantly, it is the lamellae that provides a large surface area and a short distance (short diffusion pathway) for gas exchange to occur efficiently.
As water flows over the gill filaments and lamellae oxygen diffuses down its concentration gradient the short distance between water and blood.
Carbon dioxide diffuses the opposite way down its concentration gradient.
The gills are covered by muscular flaps called the operculum which are located on the side of a fish's head.
Gills are so thin that they cannot support themselves without water, so if a fish is taken out of water the gills collapse and the fish suffocates.
A-Level Biology: Gas Exchange in Fish - The Countercurrent exchange system.
Fish ventilate their gills to maintain the gas concentration gradient.
They continuously pump their jaws and opercula to draw water in through the mouth and then force it over the gills and out through the opercular valve behind the gills. This one-way ventilation is necessary because water is denser and more viscous than air, so it cannot be contained in delicate sac-like lungs found in airbreathing animals. In the gill lamellae the blood flows towards the front of the fish while the water flows towards the back. This countercurrent system increases the concentration gradient and
increases the efficiency of gas exchange. About 80% of the dissolved oxygen is extracted from the
water.
A-Level Biology Gas exchange in insects
All terrestrial insects face the same problem:
"gas exchange verses the need to conserve water".
Effective gas exchange surfaces are large, thin and permeable it's also the same large, thin and permeable surfaces that are particularly favourable to evaporation (water loss).
So how do insects balance water loss and gas exchange.
Insects have adaptations to limit water loss - reduce evaporation of water...
-
Waterproof covering over their body surfaces. This is usually a rigid outer skeleton (exoskeleton) covered with a waterproof cuticle.
-
Small surface area to volume ratio to minimise the area over which water is lost.
-
Tiny hairs around their spiracles.
How does air get into the tracheal system?
Air passes into the trachea by way of a specialised openings in the exoskeleton called – Spiracles, which can be opened and closed by valves – preventing water loss!
Since the circulatory system of insects does not transport respiratory gases – the rhythmic ventilation (abdominal movements which moves air into and out of the spiracles) causes CO2 to move down its own concentration gradient towards the tracheoles - trachea - spiracles to be released into the atmosphere.
The Digestive System
Humans, like all animals, use Holozoic nutrition, which consists of these five stages: -
1. Ingestion – taking large pieces of food into the body
2. Digestion – breaking down the food by mechanical and chemical means
3. Absorption – taking up the soluble digestion products into the body's cells
4. Assimilation – using the absorbed materials
5. Egestion – eliminating the undigested material. (Do not confuse egestion, which is the elimination of material from a body cavity, with excretion, which is the elimination of waste material produced from within the body's cells.)
When you read “Digestion: think “Hydrolysis” - since the whole point of digestion is to break down (digest) i.e. “hydrolyse” large foods into the monomers from which they are made, so that the body can easily absorb and assimilate them! (Remember “Assimilate” means to put the monomers (products of digestion) to use, e.g. glucose is used in cellular respiration to produce ATP).
The Alimentary Canal
The human digestive system comprises a long tube called the alimentary canal (or digestive tract / gut) which extends from the mouth to the anus. In addition to the alimentary canal the digestive system is comprised of a number of associated glands (e.g. the salivary glands, the pancreas and the liver and the gall bladder).
[Insert Image of Digestive System]
You must also know that the digestive system is made up of different tissues doing different jobs.
The lining wall of the alimentary canal appears different in different parts of the gut, reflecting their different roles, but always has these four basic layers: -
1. The mucosa, which secretes digestive juices and absorbs digested food. It is often folded to increase its surface area. There is a layer of columnar epithelial cells lining the mucosa. These epithelial cells contain microvilli, membrane proteins for facilitated diffusion and active transport, mitochondria, and membrane-bound enzymes. Epithelial cells are constantly worn away by friction with food moving through the gut, so are constantly being replaced.
2. The submucosa, which contains blood vessels, lymph vessels and nerves to control the muscles. It may also contain secretory glands.
3. The muscle layer, which is made of smooth muscle, under involuntary control. It can be subdivided into circular muscle (which squeezes the gut when it contracts) and longitudinal muscle (which shortens the gut when it contracts). These two muscles therefore have opposite effects and so are antagonistic. The combination of these two muscles allows food to be pushed along the gut by peristalsis.
4. The serosa, which is a thin, tough layer of connective tissue that holds the gut together, and attaches it to the abdomen.
[insert image showing tissue layers]
Overview of the Digestive system - a journey from mouth to large intestine.
Every time you eat, the food that is masticated (chewed) in the month follows the same journey through the digestive system…
Digestion begins in the mouth.
The Mouth (Buccal cavity). The teeth and tongue physically break up the food into small pieces with a larger surface area, and form it into a ball or bolus. The salivary glands secrete saliva, which contains water to dissolve soluble substances, mucus for lubrication, lysozymes to kill bacteria and salivary amylase to digest starch. The food bolus is swallowed by an involuntary reflex action through the pharynx (the back of the mouth). During swallowing the trachea is blocked off by the epiglottis to stop food entering the lungs.
From the mouth to the Oesophagus (gullet).
Oesophagus (gullet). This is a simple tube through the thorax, which connects the mouth to the rest of the gut. No digestion takes place in the Oesophagus!.
There is an epithelium, no villi, a few glands secreting mucus, and a thick layer of circular and longitudinal muscle to propel the food (bolus) via peristalsis.
What is Peristalsis?
Peristalsis is a wave of circular muscle contraction. This Muscular contraction passes down the gut and is completely involuntary.
The oesophagus is a soft tube that can be closed. (Unlike the trachea, which is a hard tube, held open by rings of cartilage).
[insert image of peristalsis]
From Oesophagus and into the Stomach.
The Stomach is an expandable ‘bag’ where the food is stored for up to a few hours.
There are three layers of muscle to churn the food (bolus) into a liquid called chyme.
This chime is gradually released in to the small intestine via the pyloric sphincter, a region of thick circular muscle that acts as a valve.
The mucosa of the stomach wall has no villi, but does have numerous gastric pits (104 cm-2) leading to gastric glands in the mucosa layer.
These glands secrete gastric juice, which contains: -
Hydrochloric acid (pH 1): The main function of Hydrochloric acid is to to kill bacteria! The acid does not aid digestion, in fact it hinders digestion because the low pH (acidic pH 1) denatures most enzymes. Thus, Carbohydrate digestion stops and protein digestion begins.
Mucus: Mucus lubricates food and coats the epithelium to protect it from the acidic conditions of the stomach and some protease enzymes.
Only protein digestion takes place in the stomach!
From the stomach to the small intestine.
The Small Intestine is about 6.5M long, and is typically be divided into three sections: -
1. The duodenum (30 cm long).
2. The jejunum (2 m long)
3. The ileum (4 m long).
Note: Since the jejunum and ileum are similar in humans, and are the site of final digestion and all absorption (at A-level they are both typically considered as “the Ileum” i..e the rest of the small intestine after the duodenum.
The duodenum
The first 30cm of the small intestine is called the duodenum. Although this is short,
almost all the digestion takes place here, due to two secretions: pancreatic juice and bile.
Pancreatic juice is secreted by the pancreas into the duodenum through the pancreatic duct.
Pancreatic juice contains numerous amylase, protease and lipase enzymes.
Bile is secreted by the liver, stored in the gall bladder, and released into the duodenum through the bile duct.
Bile doesn’t contain any enzymes, but it does contain bile salts to aid lipid digestion, and the alkali sodium hydrogen carbonate to neutralise the stomach acid.
This alkali gives chyme in the duodenum a pH of around 7.5, so the pancreatic enzymes can work at their optimum pH.
The mucosa of the duodenum has few villi, (since there is no absorption), but the submucosa contains glands secreting mucus and sodium hydrogen carbonate.
The rest of the small intestine is called the Ileum. (jejunum and ileum).
The Ileum is the site of final digestion and absorption. There are numerous glands in the mucosa and submucosa secreting enzymes, mucus and sodium hydrogen carbonate. To maximise the rate of absorption the ileum has the three features which are dictated by Fick’s law!
1. Large Surface Area. The ileum has a huge surface area. It is over 6m long; the mucosa has large circular folds, villi and the epithelial cells have microvilli. Don't confuse these two: villi are large structures composed of hundreds of cells that can easily be seen with a light microscope, while microvilli are small subcellular structures formed by the folding of the plasma membrane of individual epithelial cells.
Microvilli can only be seen clearly with an electron microscope, and appear as a fuzzy brush border under the light microscope. The total internal surface area of the ileum is over 2000m2.
2. Short diffusion pathway. There is a network of blood capillaries in the submucosa of
each villus, so between the lumen of the gut and the blood there is just one layer of epithelium
lining the mucosa and one layer of endothelium lining the capillaries.
3. A high concentration gradient is maintained by mixing the fluids on either side of the exchange surface. On the lumen side, the circular and longitudinal muscles propel the chyme by peristalsis, and mix the contents by pendular movements (bi-directional peristalsis). The microvilli can also wave to stir the contents near the epithelial cells. On the blood side, the blood flow ensures there is always a low concentration of nutrients.
[insert images of small intestine, villi, microvilli etc.]
From the Small intestine to the Large Intestine.
The large intestine comprises the caecum, appendix, colon and rectum.
Food can spend around 36 hours in the large intestine, while water is absorbed to form semi-solid faeces.
The mucosa contains villi but no microvilli, and there are numerous glands secreting mucus. Faeces is made up of plant fibre (cellulose mainly), cholesterol, bile, mucus, mucosa cells (around 250 g of cells are lost each day!), bacteria and water, and is released by the anal sphincter.
How (and where) are Carbohydrates Digested?
By far the most abundant carbohydrate in the human diet is starch (in bread, potatoes, cereal, rice, pasta, biscuits, cake, etc).
Starch is digested (broken down / hydrolysed) into glucose in two stages: -
[insert images - flow diagram of starch-maltose-glucose]
1. the digestion (hydrolysis) of starch into the disaccharide maltose
2. the digestion (hydrolysis) of maltose into glucose.
Carbohydrate digestion begins in the mouth.
Mastication (chewing your food!) is of massive importance, since mastication helps in the production of Salivary amylase.
Salivary amylase begins the digestion of starch in the mouth. Albeit, very little digestion actually takes place, and as the chewed up food (now called a bolus) passes into the stomach, salivary amylase is quickly denatured!
However, salivary amylase in the mouth also helps to clean the mouth of bits of starch and reduce bacterial infection!
Carbohydrate digestion continues in the Duodenum.
Since no carbohydrate digestion takes place in the stomach due to amylase enzyme being denatured (low, acidic pH denatures salivary amylase enzymes). We continue carbohydrate digestion in the small intestine, specifically the duodenum.
The Pancreas secretes pancreatic amylase into the small intestine (duodenum) which digests all the remaining starch (in the duodenum).
Pancreatic Amylase digests starch molecules from the ends of the chains in two-glucose units, forming the disaccharide maltose. Glycogen is also digested here.
Disaccharides in the Ilium.
Starch has been digested (hydrolysed) into the disaccharide maltose in the beginning section of the small intestine (the duodenum). Now, these disaccharides continue their journey into the ileum where membrane bound enzymes complete the hydrolysis of maltose into glucose.
Disaccharidases in the membrane of the ileum epithelial cells complete the digestion of disaccharides to monosaccharides. This includes maltose from starch digestion as well as any sucrose and lactose in the diet.
There are three important disaccharidase enzymes you must know:
-
Maltase: Hydrolyses the disaccharide Maltose into Glucose.
-
Sucrase: Hydrolyses the disaccharide sucrose into Glucose + Fructose
-
Lactase: Hydrolyses the disaccharide Lactose into Glucose + Galactose.
(Now you should be beginning to understand why the basic biochemistry stuff is of such vital importance!)
These enzymes (maltase, sucrase and lactase) are unusual in that they are located in the membrane of the ileum epithelial cells (thus, they are membrane bound enzymes).
(Insert diagram showing membrane bound enzymes and hydrolysis).
This is an important adaptation as it means glucose can be produced at the cells where it needs to be absorbed into the body.
How is Glucose Absorbed into the body?
Glucose (and the other monosaccharides) are absorbed from the gut by a special kind of active transport called coupled active transport.
In coupled active transport the monosaccharides are transported by a facilitated diffusion protein, which is coupled to an active transport protein.
1. The active transport protein is the sodium-potassium ATPase which is present in all animal cell membranes.
This protein continuously pumps sodium ions out of the epithelial cell and potassium ions into the cell, using the energy from the hydrolysis of ATP to do so. Because this is active transport, the ions are pumped against their concentration gradients, which results in a large build-up of sodium ions in the lumen of the gut.
2. A facilitated diffusion protein called the sodium-glucose co-transporter protein, (which is only found in the membrane of the epithelial cells of the ileum), has two binding sites:
1. A binding site for glucose and
2. A binding site for sodium ions.
It is important that you know, both molecules (sodium and glucose) must be carried together.
However, since the process is diffusion, the molecules are only carried down their concentration gradients. But how, if glucose is being used (assimilated) and the concentration gradient is low for glucose? (i.e. glucose is being idffused against is concentrations gradient!)
Well, the very large concentration gradient of sodium ions across the epithelial cell membrane drives the sodium-glucose co-transporter in one direction only – carrying both molecules into the cell. So, whilst the sodium ions are diffusing down their concentration gradient, the glucose molecules can be carried across, against their concentration gradient!
3. The sodium ions are pumped out again by the Na/K ATPase to restore the sodium gradient. The potassium ions diffuse out through a potassium ion channel, doing no work in the process. So both ions constantly cycle in and out of the epithelial cell.
4. There is now a high concentration of glucose inside the epithelial cell. The glucose diffuses through the epithelial cell and diffuses into the tissue fluid of the villus via facilitated diffusion, through a glucose carrier protein that is only found on the inner surface of the epithelial cell.
5. The glucose enters the blood capillary by diffusing through gaps between the capillary endothelial cells. The glucose is carried in the blood to every cell in the body, where it is used for cellular respiration.
Remember!
The combination of steps 1 and 2 is the transport of glucose against its concentration gradient into the epithelial cell, together with the hydrolysis of ATP. So, in effect the energy released by splitting ATP is used to pump glucose into the cell, though indirectly.
Hence This is called “Coupled Active Transport”.
The Digestion of Proteins
Rennin (in gastric juice) converts the soluble milk protein caesin into its insoluble calcium salt. This keeps in the stomach longer so that pepsin can digest it. Rennin is normally only produced by infant mammals. (It is used commercially to make cheese).
Pepsin (in gastric juice) digests proteins to peptides, 6-12 amino acids long. Pepsin is an endopeptidase, which means it hydrolyses peptide bonds in the middle of a polypeptide chain. It is unusual in that it has an optimum pH of about 2 and stops working at neutral pH.
Pancreatic endopeptidases continue to digest proteins and peptides to short peptides in the duodenum. Different endopeptidase enzymes cut at different places on a peptide chain because they have different target amino acid sequences, so this is an efficient way to cut a long chain up into many short fragments, and it provides many free ends for the next enzymes to work on.
Exopeptidases in the membrane of the ileum epithelial cells complete the digestion of the short peptides to individual amino acids. Exopeptidases remove amino acids one by one from the ends of peptide chains. Carboxypeptidases work from the C-terminal end, aminopeptidases work from the N-terminal end, and dipeptidases cut dipeptides in half.
The amino acids are absorbed by active transport into the epithelial cells of the ileum, whence they diffuse into the blood capillaries of the villi. Again, the membrane-bound peptidases and the amino acid transporters are closely associated.
Protease enzymes are potentially dangerous because they can break down other enzymes (including themselves!) and other proteins in cells. To prevent this they are synthesised in the rER of their secretory cells as inactive forms, called zymogens. These are quite safe inside cells, and the enzymes are only activated in the lumen of the intestine when they are required.
Pepsin is synthesised as inactive pepsinogen, and activated by the (HCL) acid / pepsin in the stomach.
1. Rennin is synthesised as inactive pro-rennin, and activated by pepsin in the stomach
2. The pancreatic exopeptidases are activated by specific enzymes in the duodenum
3. The membrane-bound peptidase enzymes do not have this problem since they are fixed, so cannot come into contact with cell proteins.
The lining of mucus between the stomach wall and the food also protects the cells from the protease enzymes once they are activated.
[insert images of protein digestion]
[link to protein stricture and amino acids] [again an understanding of basic A-Level biochemistry is essential for answering challenging A-Level biology questions!]
The Digestion of Lipids (Triglycerides)
Digestion of Triglycerides (lipids / fats): Lipids (fats) are emulsified by bile salts to form small oil droplets called micelles, which have a large surface area!
Pancreatic lipase enzymes digest triglycerides to fatty acids and glycerol in the duodenum.
Fatty acids and glycerol are lipid soluble and diffuse across the membrane (by lipid diffusion) into the epithelial cells of the villi in the ileum.
In the epithelial cells of the ileum triglycerides are re-synthesised (!) and combine with proteins to form tiny lipoprotein particles called chylomicrons.
Chylomicrons diffuse into the lacteal - the lymph vessel inside each villus. The emulsified fatty droplets give lymph its milky colour, hence name lacteal.
Chylomicrons are carried through the lymphatic system to enter the bloodstream at the vena cava, and are then carried in the blood to all parts of the body. They are stored as triglycerides in adipose (fat) tissue.
Fats are not properly broken down until they needed / used for respiration in liver or muscle cells!
The Digestion of Nucleic acids and other substances.
Pancreatic nuclease enzymes digest nucleic acids (DNA and RNA) to nucleotides in the duodenum.
Membrane-bound nucleotidase enzymes in the epithelial cells of the ileum digest the nucleotides to sugar, base and phosphate, which are readily absorbed.
Many substances in the diet are composed of small molecules that need little or no digestion.
These include simple sugars, mineral ions, vitamins and water. Which are absorbed by different transport mechanisms, for example: -
Cholesterol and the fat-soluble vitamins (A, D, E, K) are absorbed into the epithelial cells of the ileum by lipid diffusion.
Mineral ions and water-soluble vitamins are absorbed by passive transport in the ileum
Dietary monosaccharides are absorbed by active transport in the ileum
Water is absorbed by osmosis in the ileum and colon.
Medical conditions of the digestive system you need to know.
Lactose Intolerance
Lactose is the sugar found in mammalian milk, and, as we’ve seen, lactose is digested by the disaccharidase enzyme lactase (in the membrane of the ileum epithelial cells) to glucose and galactose:
[insert diagram of : - Lactose hydrolysed by lactase into glucose + galactose]
Some people are lactose intolerant, which means they feel ill if they eat foods containing milk.
The symptoms include flatulence and explosive diarrhoea. This happens because they don’t have the lactase enzyme, so they can’t digest lactose. Since lactose can’t be absorbed it remains in the gut, passing on to the large intestine, where there wouldn’t normally be any sugars. This is where the problems are caused:
1. The presence of lactose lowers the water potential of the colon lumen, so water cannot be absorbed from the faeces by osmosis, and in fact water often diffuses out of the colon mucosa cells in to the lumen down the water potential gradient. All this excess water in the gut lumen causes diarrhoea.
2. The colon is home to large numbers of bacteria (the commensal flora), who can respire lactose and increase in number. It is the fermentation products of the bacteria which produces acids and gases such as methane and carbon dioxide. These by products of fermentation cause flatulence.
In fact most adult humans (like all other adult mammals) are to some degree lactose intolerant, and this is the “normal” state. The sugar Lactose is only found in milk (hence the term of used “milk sugar”), which is produced by the mammary glands of female mammals to feed their young.
Suckling juvenile mammals all synthesise lactase in order to digest the lactose in milk, but when they are weaned (i.e. being eating solid foods) they stop drinking milk and the gene for lactase production is switched off.
Humans are unique in that some continue to drink animal milk even as adults, at least in some human societies. These humans generally have a mutation that causes lactase to be produced throughout life, so these people are lactose tolerant and can drink milk without any ill effects.
Societies that adopted a pastoral lifestyle (farming animals), such as most Europeans, northern Indians and some Africans, are generally lactose tolerant today. The rest (many Asian, African, Native Americans and Native Australians) remain lactose intolerant as adults.
Cholera.
Cholera is an infectious disease caused by the bacterium Vibrio cholerae.
V. cholerae is a typical prokaryotic cell with a slightly curved rod shape and a single flagellum.
[insert images of Vibrio cholerae]
[Note - This topic is also often examined / linked to prokaryotic structure and function!]
The symptoms of cholera include stomach cramps, vomiting, fever and severe diarrhoea.
In severe cases up to 20 litres of water can be lost per day, and if untreated, leads to death in 75% of cholera patients.
There were several serious outbreaks of cholera in the UK in the 19th century and cholera remains a major killer of small children in developing countries (several million deaths each year). However cholera can be treated simply and cheaply.
How does cholera cause diarrhoea?
The cholera bacterium adheres to the epithelium and secretes the cholera toxin CT.
CT enters the epithelial cells and activates a chloride ion channel in the cell membrane. This causes chloride ions to diffuse out of the cells into the lumen.
The diffusion of chloride ions into the lumen lowers the water potential in the lumen of the gut.
Water is lost from cells to the lumen by osmosis, producing diarrhoea and dehydration.
[insert images of cells, and cholear and Cl- channel]
[Notice that you must fully understand osmosis - which is often examined in relation to cholera too!]
How is cholera treated? [hint oral antibiotics are no use and you often need to explain why!]
The treatment for diarrhoea was revolutionised in the 1960s, with the development of oral rehydration therapy (ORT). This simple and cheap treatment consists of drinking an oral rehydration solution (ORS) of glucose and salt (NaCl), and sometimes other ions like potassium and bicarbonate.
ORT makes use of the sodium-glucose co-transporter protein that normally absorbs glucose into the ileum epithelial cells.
1. If both Na+ and glucose are present in the lumen, they bind to the sodium-glucose co-transporter protein. Transport only works if both molecules are present, which is why salt alone is not an effective treatment. ORS contain equimolar concentrations of glucose and salt.
2. The transporter protein carries the Na+ and glucose into the cell, down their concentration gradients.
3. This lowers the water potential inside the epithelial cells. So water diffuses from the lumen into the epithelial cells by osmosis, rehydrating cells and reducing diarrhoea.
So, why cant this bacterial disease simple be given oral antibiotics? because cholera affects the water=potential of cells causing diarrhoea - thus the antibiotics would go straight through the digestive system. As such ORT are needed.
Saprophytic digestion
Fungi (link to cell structure and function of fungal cells).
How do fungi digest, absorb and assimilate?
Fungi are not consumers like animals, but are either saprophytes (decomposers), or pathogenic.
Thus, Fungi use saprophytic nutrition. fungi are saprophytes which means they do not ingest their food, but digest it extracellularly.
How?
Fungi secrete digestive enzymes (carbohydrases, proteases and lipases) into the material that surrounds them and then absorb the soluble products (sugars, amino acids, etc).
[insert image of fungi - saprophytic digestions]
Fungi are usually composed of long thin threads called hyphae. These grow quickly, penetrating dead material such as leaves, as well as growing underground throughout soil. The 'cotton wool' appearance of bread mould growing on decaying bread is typical of a mass of hyphae, called a fungal mycelium. These thin hyphae give fungi a large surface area to volume ratio. They contain many nuclei, since they are formed from the fusion of many cells.
[Think about how fungal digestion links to ecology - i.e. nutrient cycles]
The Endocrine System
Humans have two complementary control systems that they can use to respond to their environment: The Nervous system and the Endocrine (or hormonal) system.
[It is important that you are able to compare and contrast the nervous system and endocrine system]
Lets begin will a diagram of the endocrine system and a table showing the hormones, target organ and function:
[insert diagram of endocrine system w/ table]
This diagram with corresponding table shows some of the main endocrine glands and their hormones. The hormones marked with a * are ones that you’ll need to know in more detail, and will be covered in detail later.
Hormones are secreted by glands into the blood stream.
There are two kinds of glands:
1. Exocrine glands. Exocrine glands secrete solutions to the outside, or to body cavities, usually through ducts (tubes). e.g. sweat glands, tear glands, mammary glands, digestive glands.
2. Endocrine glands. Endocrine glands do not have ducts but secrete chemicals directly into the tissue fluid, and subsequently these ‘chemicals’ diffuse into the blood stream. The hormone secreting glands are all endocrine glands, e.g. thyroid gland, pituitary gland, and adrenal gland.
Once a hormone has been secreted by its gland, it diffuses into the blood stream and is carried around the body to all organs. However, specific hormones only affect certain ‘target organs’, which can respond to it.
Target organs have specific receptor molecules in their cells to which the hormone binds. The receptor molecules are proteins and they form specific hormone-receptor complexes, in very much the same way enzyme-substrate complexes are formed.
Cells without the specific receptor will just ignore a hormone.
The hormone - receptor complex can affect almost any aspect of a cell’s function, including metabolism, transport, protein synthesis, cell division or cell death.
There are three different ways in which a hormone can affect cell function: -
1. Some hormones affect the permeability of the cell membrane. They bind to a receptor on the membrane, which then activates a transporter, so substances can enter or leave the cell. (E.g. acetylcholine stimulates sodium uptake.)
2. Some hormones release a “second messenger” inside the cell. They bind to a receptor on the membrane, which then activates an enzyme in the membrane, which catalyses the production of a chemical in the cytoplasm, which affects various aspects of the cell. (E.g. adrenaline stimulates glycogen breakdown.)
3. The steroid hormones are lipid-soluble so can easily pass through membranes by lipid diffusion. They diffuse to the nucleus, where they bind to a receptor, which activates protein synthesis. (E.g. testosterone stimulates spermatogenesis.)
[insert images showing example of 1, 2 and 3 above]
In most cases, hormones do not enter into cells, rather the effect of a hormone is determined not by the hormone itself, but by a receptor on/in the target cell. Thus, the same hormone can have different effects in different target cells!
As stated above it is important that you are able to compare and contrast the endocrine system and nervous system. Moreover, you must understand that these systems work closely together, e.g. endocrine glands are usually controlled by the nervous system, and a response to a stimulus often involves both systems.
Table comparing Nervous and Endocrine (hormone) systems:
[insert table]
What is Paracrine Signalling?
Paracrine signalling is communication between close cells using chemicals called local chemical mediators.
These chemicals are released by cells into the surrounding tissue fluid, but not into the blood. Thus they only have a local effect on the cells surrounding their release, in contrast to hormones. Like hormones and neurotransmitters they bind to receptors on the surface of the target cells to cause an effect. Two such local mediators are prostaglandins and histamine, which we’ll consider next.
What are Prostaglandins?
Prostaglandins are lipid molecules.
Prostaglandins are lipid molecules that are produced by cells in almost every tissue in the body, targeting local smooth muscle cells and endothelial (lining cells). There are over a dozen different prostaglandins known, causing a number of effects, especially the inflammatory response to injury and infection. Prostaglandins cause vasodilation by stimulating smooth muscle cells in the walls of local arterioles to relax, increasing blood flow to the area (so the area turns red). Prostaglandins stimulate the blood clotting process (so wounds are sealed) and Prostaglandins stimulate the pain (receptors) neurones.
Did you know that Anti-inflammatory drugs, e.g. aspirin and ibuprofen, reduce inflammation and associated pain by inhibiting a key enzyme in the synthesis of prostaglandins.
What is Histamine?
You’re most likely aware that histamines are also involved in the inflammatory response, e.g. inflammation following stings from insects or nettles but what is histamine?
Histamine is compound made from the amino acid histidine, which is stored in granules in mast cells (found in connective tissue, especially in the skin).
When stimulated by the immune system or by injury, mast cells release histamine into the surrounding tissue fluid. Here, Histamine stimulates vasodilation of nearby arterioles, loosening of nearby capillary walls, which in turn causes capillaries to leak. The leaking of capillaries allows blood plasma and leukocytes (white blood cells) to reach the site injury.
Histamine also causes broncho-constriction of the airways (the bronchi). In fact, sometimes too much histamine can be released, which results in an “allergic reaction” specifically this is an extreme inflammatory response due to too much histamine. As such, antihistamine drugs inhibit the release of histamine and so are used to counter allergic reactions.
Note: The action of neurotransmitters at synapses can also be described as paracrine signalling, since these chemicals only have a local effect and are not released into the blood.
Homeostasis
Homeostasis literally means “standing still” and it refers to the process of keeping the internal body environment in a steady state. The importance of this cannot be over-stated since a great deal of the hormone system and autonomic nervous system is dedicated to homeostasis.
Factors that are controlled by homeostasis include: -
Body temperature – to keep enzymes working near their optimum temperature and stop them denaturing
Blood pH – to keep enzymes working near their optimum pH
Blood (sugar) glucose concentration – to ensure there is enough glucose available for cellular respiration, but not enough to lower the blood water potential and dehydrate cells
Blood water potential – to prevent loss or gain of water from cells by osmosis
You’ll need to know two examples of homeostasis in detail: temperature and blood glucose (but be aware that you may have to apply knowledge of homeostatic mechanisms to the others too!)
Negative and positive feedback
All homeostatic mechanisms use “Negative Feedback” to maintain a constant value (known as the set point).
Negative feedback means that whenever a “change” occurs in a system, the change automatically causes a corrective mechanism to start. This corrective course of action reverses the “change” and brings the system back to normal (i.e. back to its set point).
[insert negative feedback graph]
So, in a system controlled by negative feedback the level is never maintained perfectly, but constantly oscillates about the set point. An efficient homeostatic system minimises the size of the oscillations.
Temperature Homeostasis (Thermoregulation)
One of the most important examples of homeostasis is the regulation of body temperature. There are basically two ways of doing this: mammals and birds can generate their own heat and are called endotherms; while all other animals rely on gaining heat from their surroundings, so are called ectotherms.
Temperature Homeostasis in Endotherms
Endotherms (mammals and birds) can generate heat internally, have thermal insulation, and can usually maintain a remarkably constant body temperature. Humans and most other mammals maintain a set point of 37.5 ± 0.5 °C, while birds usually have set points of 40-42°C. Endotherms are sometimes called warmblooded animals, but this term isn’t very scientific, partly because endotherms can get quite cold (e.g. during hibernation). Endotherms don’t keep their whole body at the same temperature: they maintain a constant core temperature; while allowing the peripheral temperature to be colder, especially the surface, which is in contact with the surroundings.
The advantage of being endothermic is that animals can survive in a wide range of environmental temperatures, and so can colonise almost any habitat, and remain active at night and in cold weather.
This gives endothermic predators an obvious advantage over ectothermic prey. The disadvantage is that it requires a lot of energy, so endotherms need to eat far more than ectoderms.
In humans temperature homeostasis is controlled by two thermoregulatory centres in the hypothalamus.
The thermoregulatory centres receive input from two sets of thermoreceptors: receptors in the hypothalamus itself monitor the temperature of the blood as it passes through the brain (the core temperature), and receptors in the skin monitor the external temperature. Both pieces of information are needed so that the body can make appropriate adjustments.
One of the thermoregulatory centres – the heat loss centre – is activated when the core temperature rises. It sends impulses to several different effectors in the body to reduce the core temperature.
The other thermoregulatory centre – the heat gain centre – is activated when the core temperature falls. It sends impulses to several different effectors in the body to increase the core temperature.
The thermoregulatory centre is part of the autonomic nervous system, so the various responses are all involuntary. The exact responses to high and low temperatures are described in the table below:
[insert table]
Note that some of the responses to low temperature actually generate heat (thermogenesis), while others just conserve heat.
Similarly some of the responses to cold actively cool the body down, while others just reduce heat production or transfer heat to the surface.
Therefore, the body has a range of responses available, depending on the internal and external temperatures.
[insert diagram of thermoregulatory centres]
Mammals can alter their set point in special circumstances:
Fever. Chemicals called pyrogens released by white blood cells raise the set point of the thermoregulatory centre causing the whole body temperature to increase by 2-3 °C. This helps to kill bacteria and explains why you shiver even though you are hot.
Hibernation. Some mammals release hormones that reduce their set point to around 5°C while they hibernate. This drastically reduces their metabolic rate and so conserves their food reserves.
Torpor. Bats and hummingbirds reduce their set point every day while they are inactive. They have a high surface area: volume ratio, so this reduces heat loss.
Failure of temperature homeostasis
Hypothermia occurs when heat loss exceeds heat generation, due to prolonged exposure to cold temperatures. As the core temperature decreases the metabolic rate also decreases, leading to less thermogenesis. If the core temperature drops below 32°C shivering stops so the core temperature drops even further. If the core temperature falls below 30°C hypothermia is usually fatal.
Hyperthermia occurs when heat gain exceeds heat loss, usually due to prolonged exposure to high temperatures. This situation is often associated with dehydration, which reduces sweating, the only effective way to cool down. A rise in core temperature increases the metabolic rate, fuelling a further increase in temperature. If the core temperature rises above 40°C hyperthermia is usually fatal.
Both hypothermia and hyperthermia are examples of the dangers of positive feedback.
Positive feedback occurs when the change stimulates a further change in the same direction. Positive feedback is potentially dangerous and is usually associated with a breakdown in the normal control mechanism.
[insert positive feedback graph]
Temperature Control in Ectotherms (link to evolution adaptations)
Ectotherms (all animals except mammals and birds) rely on external heat sources to warm up; do not have thermal insulation; and their body temperature varies with the environmental temperature.
Ectotherms are sometimes called cold-blooded animals, but this term isn’t very scientific, because ectoderms can get very warm. Reptiles, such as lizards, iguanas and crocodiles are classic ectotherms. They cannot warm up by shivering because, if their temperature is low, they cannot respire fast enough to make ATP for rapid muscle contraction. Instead, reptiles regulate their body temperature by thermoregulatory behaviour e.g: -
Iguanas start every day by basking on rocks in the sun until their metabolic rate is fast enough for them to become active.
Lizards lie down on warm ground to gain heat, and raise themselves off the ground if they get too hot.
To prevent overheating in the midday sun lizards take shelter under rocks or vegetation.
Some lizards can adjust the amount of heat they gain by changing their angle to the sun. Turning their backs to the sun presents the maximum surface area, while pointing towards the sun presents the minimum surface area.
Crocodiles can move between the land and the water during the day to maintain a constant temperature.
At night, lizards shelter in burrows, which provide insulation to reduce heat loss (and hide them from predators).
The advantage of being ectothermic is that animals use far less energy than endoderms. At rest the metabolic rate of a reptile is only 10% of that of a mammal of similar size. At night, when the core temperature of ectotherms drops with the temperature of the surroundings, their metabolic rate drops still further. This means ectothermic animals need to eat far less than endotherms, and can often survive for weeks without eating. The disadvantages are that, at certain times of the day, ectotherms can only move slowly and have slow reactions. This makes them easy prey and poor predators.
Blood Glucose Homeostasis
Glucose is the transport carbohydrate in animals, and its concentration in the blood affects every cell in the body. The brain in particular can only respire glucose (not lipids) but it doesn’t store glycogen. Very low concentrations of glucose (hypoglycaemia) will cause brain cells to die and very high concentrations (hyperglycaemia) will lower the blood water potential and kill cells by dehydration. The concentration of glucose in the blood is therefore strictly controlled within the range 80-100 mg 100cm-3.
[insert diagram shows the main sources and fates of blood glucose]
The main source of blood glucose is the digestion and absorption of dietary carbohydrate (mostly starch). Blood from the intestine goes directly to the liver in the hepatic portal vein before being carried to the rest of the body.
Glucose is mainly used for respiration in all cells. It can also be used to synthesise amino acids, nucleotides, etc.
For storage glucose can be converted to the polysaccharide glycogen in liver and muscle cells (glycogenesis). This is reversible, and when glucose is needed the glycogen can be broken down again (glycogenolysis).
Excess glucose can also be converted to triglycerides in the liver (lipogenesis) then transported as lipoproteins to adipose tissue for storage. This process is irreversible: triglycerides can be used in aerobic respiration but cannot be used to make glucose.
Animals don’t normally synthesise glucose, but when dietary glucose is scarce proteins and nucleic acids can be used to synthesise glucose (gluconeogenesis).
Control of blood glucose concentration
Blood glucose concentration is unusual in that it is not controlled by the CNS, but by the pancreas, which is both an exocrine and an endocrine organ. Regions of the pancreas, called the islets of Langerhans, serve as both glucose receptors and as endocrine cells, releasing hormones to effect the control of glucose.
There are two kinds of islet cells, called alpha and beta cells. Both cells have glucose receptors, but the alpha cells detect low glucose concentrations and respond by secreting glucagon, while the beta cells detect high glucose concentrations and respond by secreting insulin.
These two hormones are antagonistic, which means that they have opposite effects on blood glucose.
[insert glucose feedback diagram]
After a meal, glucose is absorbed from the gut into the bloodstream, increasing the blood glucose concentration. This increase is detected by the pancreas, which secretes insulin from its beta cells in response.
Insulin causes glucose to be taken up by the liver and converted to glycogen. This reduces blood glucose, which is detected by the pancreas, which stops secreting insulin. If the glucose level falls too far, the pancreas detects this and releases glucagon from its a cells. Glucagon causes the liver to break down some of its glycogen store to glucose, which diffuses into the blood. This increases blood glucose, which causes the pancreas to stop producing glucagon. These negative feedback loops continue all day, as shown in this graph:
[insert graph]
The mechanism of glucose homeostasis hormone action
[insert diagram]
This diagram summarises how the hormones insulin and glucagon exert their effects. It also includes the hormone adrenaline, which is the “fight or flight” hormone released by the adrenal glands. Amongst many other effects, adrenaline also stimulates the release of glucose from the liver, to provide more energy for muscle contraction.
1. Insulin molecules bind to an insulin receptor protein in the cell membrane. This binding activates an enzyme active site on the inner surface of the same membrane protein, which catalyses a reaction activating a molecule called insulin receptor substrate (IRS). IRS is the second messenger for insulin.
2. IRS has two separate effects in the cell. Firstly it increases the rate of glucose uptake by recruiting more glucose transporters to the cell membrane. These receptors are stored in cytoplasmic vesicles, and IRS causes these vesicles to fuse with the cell membrane.
3. Secondly, IRS actives the enzyme glycogen synthase, which synthesises glycogen from cytoplasmic glucose. In fact there are several steps here: IRS activates one enzyme, which activates another, which activates another, which activates glycogen synthase. This cascade amplifies the effect, so each molecule of insulin can activate thousands of molecules of glycogen synthase.
4. If present in the blood, glucagon and adrenaline each bind to their specific membrane receptor proteins.
5. These hormone-receptor complexes now activate a membrane-bound enzyme called adenylate cyclase, which catalyses the conversion of ATP to cAMP (cyclic adenosine monophosphate), which is the second messenger for these hormones.
6. cAMP activates the enzyme glycogen phosphorylase, which catalyses the breakdown of glycogen to glucose. This process involves another multi-step cascade amplification.
These hormones demonstrate two advantages of the second messenger system: one hormone (insulin in this case) can have two completely different effects in a cell; while two different hormones (glucagon and adrenaline in this case) can have the same effect in a cell. Each hormone can have different effect in different cell types too.
Diabetes Mellitus
Diabetes is a disease caused by a failure of glucose homeostasis.
There are two forms of the disease.
In insulin-dependent diabetes (also known as IDDM, type 1 or early-onset diabetes) there is a severe insulin deficiency due to autoimmune killing of b cells (possibly due to a virus). This type usually appears in childhood.
In non insulin-dependent diabetes (also known as NIDDM, type 2 or late-onset diabetes) insulin is produced, but the insulin receptors in the target cells don’t work, so insulin has no effect. This type tends to appear in overweight people at around age 40, and it accounts for 90% of diabetes cases in the industrialised world.
In both cases there is a very high blood glucose concentration after a meal, so the kidney can’t reabsorb it all back into the blood, so much of the glucose is excreted in excess urine (diabetes mellitus means “sweet fountain”). This leads to the symptoms of diabetes:
• high thirst due to osmosis of water from cells to the blood, which has a low water potential.
• copious urine production due to excess water in blood.
• poor vision due to osmotic loss of water from the eye lens.
• tiredness due to loss of glucose in urine and poor uptake of glucose by liver and muscle cells, so no glycogen stores.
• muscle wasting due to gluconeogenesis caused by increased glucagon.
Until the discovery of insulin in 1922 by Banting and Best, diabetes was an untreatable, fatal disease. Today diabetes can be treated by injections with insulin or by careful diet. Insulin can be extracted from the pancreas tissue of cattle and pigs, or it can be made in fermenters by genetically-engineered bacteria (pxx).
Treatment is improving all the time, with the development of fast-acting and slow-acting insulin preparations; simpler injection pens; oral insulin preparations (insulin, a protein hormone, is normally digested in the intestine); portable insulin infusion pumps; and even the possibility of islets of Langerhans transplants.
Control of the Mammalian Oestrous Cycle
Female mammals produce eggs and become receptive to mating in a regular cycle, called the oestrus cycle.
The oestrus cycle can vary in length from five days in mice to one year for red deer. Oestrus itself refers to the period of the cycle when the female is sexually receptive, or “on heat”. For example dogs usually come into heat twice a year. In humans the oestrus cycle is also known as the menstrual cycle because it is one month long (from the Latin mens, month). Humans (and other primates) are unusual in that the females are sexually receptive throughout the year, and the uterine lining (the endometrium) is shed each month through the vagina in the process of menstruation (the “period”).
The menstrual cycle in humans is controlled by four hormones secreted by two glands.
• The pituitary gland, below the hypothalamus in the brain, secretes the hormones follicle stimulating hormone (FSH) and luteinising hormone (LH), which target the ovaries.
• The ovaries are endocrine organs as well as creating and releasing ova. They secrete the hormones oestrogen and progesterone, which target the pituitary gland and the uterus.
The effects of these four hormones are shown in this diagram.
[insert diagram]
Each hormone affects the release of other hormones by negative and positive feedback loops, so each hormone is produced in sequence. This chart shows how the concentration of each hormone in the blood changes throughout a 28-day cycle.
[insert graph]
1. FSH is secreted by the pituitary glands, and stimulates the development of a Graafian follicle in one of the ovaries. This follicle contains a single ovum cell surrounded by other calls.
2. The follicle secretes oestrogen, which stimulates the uterus to rebuild the endometrium wall that has been shed during menstruation. Oestrogen also affects the pituitary gland, initially inhibiting the release of FSH. However, as the follicle gradually develops, the concentration of oestrogen in the blood rises, and it starts to stimulate the release of FSH and LH by the pituitary gland.
3. The sudden surge of LH at about day 14 causes the fully developed follicle to burst, release the ovum in the oviduct – ovulation. LH also stimulates the follicle to develop into a body called the corpus luteum, which secretes progesterone.
4. Progesterone stimulates the uterus to complete the development of the endometrium wall, which is now ready to receive an embryo. Progesterone also inhibits the release of LH and FSH by the pituitary gland, which in turn stops the release of oestrogen and progesterone by the ovaries.
5. The corpus luteum degenerates over the next 10 days, due to prostaglandins produces by the ovary, so less progesterone is secreted. When the concentration of progesterone drops low enough, menstruation is triggered. The inhibition of the pituitary gland is also removed, so FSH starts to be released and the cycle starts again.
If an egg is fertilised and the embryo implants in the uterus, the embryo secretes a hormone called human chorionic gonadotrophin (HCG). HCG stops the corpus luteum degenerating, so progesterone continues to be produced and there is no menstruation. Progesterone also stops the pituitary releasing FSH, so no more ova are matured during pregnancy. Pregnancy test kits test for the presence of small amounts of HCG in the urine.
★ AQA A Level Biology Specification Reference: - 3.3.2 Gas exchange in the tracheal system of an insect (tracheae, tracheoles and spiracles).
★ Edexcel A Level Biology (Biology A – Salters-Nuffield) Specification Reference: - None Specific but useful for: Know the properties of gas exchange surfaces in living organisms (large surface area to volume ratio, thickness of surface, difference in concentration).
★ Edexcel A Level Biology (Biology B) Specification Reference: - Topic 4: Exchange and Transport: 4.3 Gas exchange: i Understand how insects are adapted for gas exchange. 7: Dissect an insect to show the structure of the gas exchange system.
★ OCR A Level Biology (Biology A) Specification Reference: - Module 3: Exchange and transport: In this module, learners study the structure and function of gas exchange and transport systems in a range of animals and in terrestrial plants. Ventilation and gas exchange systems in mammals, bony fish and insects are used as examples of the properties and functions of exchange surfaces in animals. To include: insects – spiracles, trachea, thoracic and abdominal movement to change body volume, exchange with tracheal fluid.
★ WJEC A Level Biology Specification Reference: -Component 3: REQUIREMENTS FOR LIFE: (d) the common features of the specialised respiratory surfaces of larger animals and the adaptation of respiratory surfaces to environmental conditions - the adaptations of the insect tracheal system to life in a terrestrial environment.
★ AQA A Level Biology Specification Reference: -3.3.2 Gas exchange by the leaves of dicotyledonous plants (mesophyll and stomata). Students could use an optical microscope to examine vertical sections through a dicotyledonous leaf.
★ CIE A Level Biology Specification Reference: - 7.1 Structure of transport tissues: draw and label from prepared slides plan diagrams of transverse sections of stems, roots and leaves of herbaceous dicotyledonous plants using an eyepiece graticule to show tissues in correct proportions. Draw and label from prepared slides the cells in the different tissues in roots, stems and leaves of herbaceous dicotyledonous plants using transverse and longitudinal sections.
★ Edexcel A Level Biology (Biology A – Salters-Nuffield) Specification Reference: - None Specific but useful for: 4.7 Know the ultrastructure of plant cells (cell walls, chloroplasts, amyloplasts, vacuole, tonoplast, plasmodesmata, pits and middle lamella) and be able to compare it with animal cells.
★ Edexcel A Level Biology (Biology B) Specification Reference: - Topic 4: Exchange and Transport: 4.3 Gas exchange: Understand gas exchange in flowering plants, including the role of stomata, gas
exchange surfaces in the leaf and lenticels.
★ OCR A Level Biology (Biology A) Specification Reference: - Module 3: Exchange and transport: In this module, learners study the structure and function of gas exchange and transport systems in a range of animals and in terrestrial plants. 3.1.3 Transport in plants: (b) (i) the structure and function of the vascular system in the leaves of herbaceous dicotyledonous plants.
★ OCR A Level Biology (Biology B) Specification Reference: -2.2.4 Transport systems in plants: the structure & function of leaves. To include xylem vessels, sieve tube elements and companion cells in the roots, stems and leaves of monocotyledonous crop plants (cereals) and dicotyledonous crop plants (broad-leaved crops e.g. carrots, potatoes).
★ WJEC A Level Biology Specification Reference: - Requirements for Life: 1. Adaptations for gas exchange. The role of the leaf as an organ of gas exchange, including stomatal opening and closing. SPECIFIED PRACTICAL WORK. Scientific drawing of a low power plan of a prepared slide of T.S. dicotyledon leaf e.g. Ligustrum (privet), including calculation of actual size and magnification of drawing.
★ AQA A Level Biology Specification Reference: - 3.3.2 Gas exchange across the gills of fish (gill lamellae and filaments including the counter-current principle).
★ Edexcel A Level Biology (Biology A – Salters-Nuffield) Specification Reference: - None Specific but useful for 1.1 Understand why many animals have a heart and circulation (mass transport to overcome limitations of diffusion in meeting the requirements of organisms). 2.1 i) Know the properties of gas exchange surfaces in living organisms (large surface area to volume ratio, thickness of surface, difference in concentration).
★ Edexcel A Level Biology (Biology B) Specification Reference: - Topic 4: Exchange and Transport: ii Understand why organisms need a mass transport system and specialised gas exchange surfaces as they increase in size. 4.3 Gas exchange: Understand how fish are adapted for gas exchange.
★ OCR A Level Biology (Biology A) Specification Reference: -
Module 3: Exchange and transport: In this module, learners study the structure and
function of gas exchange and transport systems in a range of animals and in terrestrial plants. Ventilation and gas exchange systems in mammals, bony fish and insects are used as examples of the properties and functions of exchange surfaces in animals. Learners should be able to demonstrate and apply their knowledge and understanding of: (f) the mechanisms of ventilation and gas exchange in bony fish bony fish – changes in volume of the buccal cavity and the functions of the operculum, gill filaments and gill lamellae (gill plates); countercurrent flow.
★ WJEC A Level Biology Specification Reference: -Component 3: REQUIREMENTS FOR LIFE: (d) the common features of the specialised respiratory surfaces of larger animals and the adaptation of respiratory surfaces to environmental conditions - fish have gills for aquatic environments and mammals have lungs for terrestrial environments. (f) ventilation in bony fish and comparison of counter current flow with parallel flow.
★ AQA A Level Biology Specification Reference: - 3.3.2 Gas exchange across the gills of fish (gill lamellae and filaments including the counter-current principle).
★ Edexcel A Level Biology (Biology A – Salters-Nuffield) Specification Reference: - None Specific but useful for 1.1 Understand why many animals have a heart and circulation (mass transport to overcome limitations of diffusion in meeting the requirements of organisms). 2.1 i) Know the properties of gas exchange surfaces in living organisms (large surface area to volume ratio, thickness of surface, difference in concentration).
★ Edexcel A Level Biology (Biology B) Specification Reference: - Topic 4: Exchange and Transport: ii Understand why organisms need a mass transport system and specialised gas exchange surfaces as they increase in size. 4.3 Gas exchange: Understand how fish are adapted for gas exchange.
★ OCR A Level Biology (Biology A) Specification Reference: -
Module 3: Exchange and transport: In this module, learners study the structure and
function of gas exchange and transport systems in a range of animals and in terrestrial plants. Ventilation and gas exchange systems in mammals, bony fish and insects are used as examples of the properties and functions of exchange surfaces in animals. Learners should be able to demonstrate and apply their knowledge and understanding of: (f) the mechanisms of ventilation and gas exchange in bony fish bony fish – changes in volume of the buccal cavity and the functions of the operculum, gill filaments and gill lamellae (gill plates); countercurrent flow.
★ WJEC A Level Biology Specification Reference: -Component 3: REQUIREMENTS FOR LIFE: (d) the common features of the specialised respiratory surfaces of larger animals and the adaptation of respiratory surfaces to environmental conditions - fish have gills for aquatic environments and mammals have lungs for terrestrial environments. (f) ventilation in bony fish and comparison of counter current flow with parallel flow.